All published articles of this journal are available on ScienceDirect.
A Review Article on Vaccine Development and Therapeutics Approach Against SARS-CoV-2
Abstract
In December 2019, a novel coronavirus (COVID-19) unleashed an unprecedented and unanticipated pandemic, causing widespread concern. More than three million deaths have been documented since the first incidence of COVID-19 discovered in China. Several arduous efforts have been made by the governments of various countries worldwide to prevent and control the SARS-CoV-2 infection. This review article discusses an update on all kinds of therapeutic interventions currently applied or developed to treat SARS-CoV-2 condition, including the repurposing of drugs such as Remdesivir, Favipiravir, Ivermectin, etc. We also discuss CRISPR’s potential involvement in antiviral therapy, convalescent plasma therapy, and immunomodulators in combination to tackle the cytokine storms and present a comprehensive overview on many vaccines that have been created to date or are under trials, as well as their platforms and efficacy. Moreover, this article also discusses the mechanism of action of every therapeutic intervention.
1. INTRODUCTION
SARS-CoV-2, referred to as severe acute respiratory syndrome corona virus-2, was first detected in Wuhan city, Hubei province, China, and it has since caused a worldwide pandemic. At the beginning (December 2019), pneumonia of unknown cause was detected, later, it was classified as novel severe acute respiratory syndrome coronavirus 2 (SARS-CoV-2) causing COVID-19. This infectious respiratory illness soon developed into a local epidemic in December 2019 and then spiraled out of control into a global pandemic since the start of 2020. SARS-CoV-2 has unequivocally been accepted as the most dangerous human pathogen displacing the Influenza H1NI, human immunodeficiency virus (HIV), and Ebola in terms of the number of casualties in a relatively shorter span. As it happens, “COVID-19 is ten times deadlier than Swine Flu,” as recently announced by Chief Dr. Tedros Adhanom Ghebreyesus of the World Health Organization (WHO).
On 30th January 2020, WHO declared COVID-19 as a global health emergency and declared it a pandemic on 11th March 2020. Given the magnitude of the disease and its potential of severe morbidities and high mortality, WHO announced the pandemic as the “defining global health crisis of our time. The distribution of deaths, recoveries, and active cases in highly affected countries globally has presented an enormous variation [1]. SARS-CoV-2 is a zoonotic disease-causing enveloped virus, having a linear single-stranded positive-sense RNA genome of about 30kb in size. It has originated in animals but can cause respiratory diseases in humans [2]. The first Human coronavirus (HCV) was recorded in the 1960s, since then more HCV strains have been discovered that caused respiratory diseases of various severities [3, 4]. Coronaviruses belong to the family of Coronaviridae, a member of the order Nidovirales, and have four genera -α, β, γ, and δ based on their phylogenetic relationships and genomic structures. The major three pathogenic species, including SARS-CoV, MERS-CoV, and SARS-CoV-2, can infect humans and belong to the β coronavirus genus. In contrast, HCV-229E and HCV-NL63 also infect humans but are a part of the α genus [4]. SARS-CoV-2 has a similar genomic structure to other beta coronaviruses, containing 14 open reading frames.
Based on genetic relationships, coronavirus is classified into (i) severe acute respiratory syndrome coronavirus (SARS-CoV), (ii) Middle East respiratory syndrome coronavirus (MERS-CoV), and (iii) SARS-CoV-2. Coronaviruses have crossed the species barrier to cause deleterious pneumonia in humans since the beginning of the 21st century. SARS-CoV-2 is associated with an ongoing outburst of atypical pneumonia [5, 6]. MERS-COV have originated in bats, but the reservoir host is feeding spillover to people through dromedary camels [6]. Both SARS-CoV and SARS-CoV-2 are closely related based on their sequence similarity and reported in bats which most likely serve as a reservoir host for these two viruses. While palm civets and raccoon dogs are the intermediate hosts for zoonotic transmission of SARS-CoV between bats and humans [7], the SARS-CoV-2 intermediate remains unclear. Symptoms associated with COVID-19 disease induced by SARS-CoV-2 infection in humans vary from asymptomatic or mild to severely ill. They may arise within two days to two weeks post-exposure to the virus. Some common symptoms are fever, cough, chest congestion, shortness of breath, fatigue, loss of taste or smell, nausea, and diarrhea. While malaise, respiratory distress, and sputum production are less common, pneumonia presents itself as one of the most severe and adverse indications of COVID-19. There is sameness in terms of clinical manifestations in SARS-CoV-2, SARS-CoV, and MERS-CoV infections. All are associated with cytokine storm build-up, where the pro-inflammatory cytokine level rises and causes complications in the infected person [8]. However, variation occurs in terms of disease spread. While both SARS and MERS are believed to be nosocomial, SARS-CoV-2 is broadly transmitted in the population [9]. The primary mode of transmission for COVID-19 is via the formation of respiratory droplets from an infected person. Their airborne transmission is the leading cause of rapid infection among the population. Here, microbes are said to be present inside the nuclei of the droplets. These particles are less than 5μm in diameter, can travel a distance greater than 1m, and remain in the air for a longer duration than respiratory droplets. Transmission by respiratory droplets can occur when the least distance between a healthy and infected individual is less than 1m which happens when the droplets released by the infected person while coughing and sneezing come in contact with a healthy person through their mucosa (mouth or nose) or conjunctiva (eyes). Thus, direct physical contact with an affected individual or their immediate environment is assumed to be a significant transmission mode. The duration to which a virus can survive on a surface cannot be determined accurately, while the approximate window ranges from 24 to 72 hours. In the case of COVID-19, it has been observed that airborne transmission might also be possible under certain exceptional circumstances and settings, e.g., in procedure or support treatments that may generate aerosols. Some evidence suggests that in cases of COVID-19, where the virus can penetrate deeper to cause intestinal infection, it may transmit through the fecal matter [10]. Studies have supported that containment measures led to the slowing of transmission of COVID-19. Social distancing adopted by some countries helped decrease the number of positive confirmed cases on a daily level. Social distancing or physical distancing refers to maintaining space and avoiding direct contact with people outside the home to limit transmission. Indeed, the lockdown has successfully slowed down the spread of COVID-19 to some extent; however, challenges are many: flouting of lockdown norms, limited testing, the vulnerability of healthcare workers, and the missing frontline support of trained researchers. Given the magnitude of the disease and non-stop increasing morbidities with high mortality rates, the whole world is waiting for the methods to prevent SARS-CoV-2 and cure its infection. Preventive strategies, proper and fast diagnosis, and available treatment options are being employed [11]. Alongside these measures, medicines and vaccines are being tested and developed [12, 13]. This review article first briefly describes the lifecycle of SARS-CoV-2, then focuses on covering the powerful drugs and in-hand therapeutic options and potential strategies which have been developed or are in development to fight against COVID-19.
2. SARS-CoV-2 LIFE CYCLE
A coronavirus virion (an infectious virus particle) goes through a replication life cycle within a host cell, making more copies that can eventually infect more cells. The critical stages of a general coronavirus replication life cycle include binding to a cell surface receptor on host cells, following cell entry, virion uncoating, RNA transcription, RNA synthesis, translation of replicase proteins, virion assembly, and release of mature virions into the extracellular space. The coronavirus virion is typically an enveloped particle surrounded by a protein capsid, as schematically shown in Fig. (1). The capsid comprises spike (S), envelope (E), membrane (M), and nucleocapsid (N) proteins, containing the single-stranded RNA genome. The S, E, M, and N proteins are all structural proteins.
Furthermore, the RNA molecule is a “plus-strand RNA” with positive polarity, 28 to 32 kilobases (kb) in length, and translated directly into protein. The distinctive “crown” appearance of coronaviruses seen under electron microscopy is due to spike proteins determining cell tropism and host species specificity. The trimeric spike proteins first attach to specific surface receptors in the host cell membrane. The interaction of spike proteins with the virus's receptor facilitates its entry to the host cell via angiotensin-converting enzyme 2 (ACE2) [14]. Upon binding, SARS-CoV-2 and host cellular membrane fuses, facilitated by a spike activation via proteolytic cleavage by a specific protease such as cathepsin or TMPRR2 and enters into the cell through endocytosis, where the enveloped receptor-bound virus enters the cytosol within a vesicle [15]. After cell entry, the viral RNA genome is released into the cytoplasm, followed by the RNA's un-coating and translating its ORF1 gene, which constitutes large overlapping of ORF1a and ORF1ab. A ribosome frame shifting sequence is present at the end of the ORF1a, consisting of an RNA pseudoknot that causes co-translation of two polyprotein precursors, pp1a and pp1ab. Pp1a consists of non-structural protein (nsp) 1-11 and pp1ab contains the complete translated coding region of the non-structural protein (nsp) 1-16. SARS-CoV-2 proteolytically processes these polyproteins encoded proteases to produce fully matured and functionally active replication machinery. These translated products obtained from proteolytic processing can regulate host cell factors and facilitate and prepare the cell for RNA synthesis via replication complex formation. The C-terminal translation product of polyprotein 1ab governs the process of replication and transcription directed by RdRp. The RdRp mediates full-length negative-strand RNA production, which later serves as a template for positive-strand virion genomic RNA.
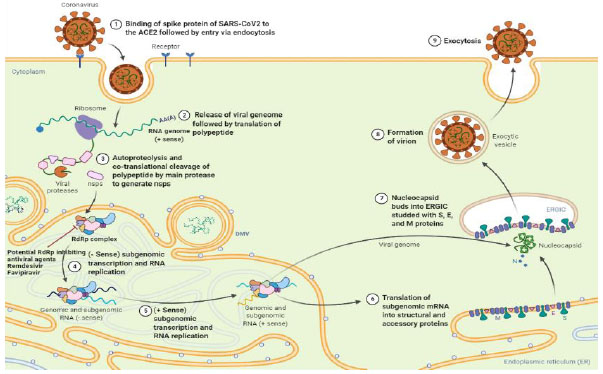
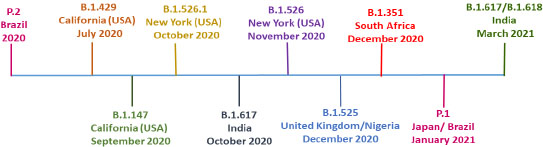
In contrast to plus-strand RNA, negative-strand RNA is complementary to the mRNA and cannot translate directly. It needs to be converted to plus-strand RNA by RNA polymerase first. The structural proteins such as spike protein (S), Membrane protein (M), Nucleocapsid protein (N), and Envelope protein (E),) are transcribed from full-length positive-strand sub-genomic RNAs [16-18]. These nonstructural and structural proteins move to assembly sites at a transitional zone between the endoplasmic reticulum (ER) and the Golgi apparatus. The Golgi apparatus and the ER are organelles involved in protein synthesis, post-translational modification of proteins, protein packaging into membrane-bound vesicles, and protein transport. After maturation in the Golgi body, the S, E, and M structural proteins combine with the nucleocapsid for the new virions to assemble and bud off from the Golgi membranes as vesicles. These vesicles are translocated to the host cell membrane, fusing with the host cell membrane and extracellular space. This process facilitates the spread of the virus by avoiding neutralization via SARS-CoV-2 specific antibodies.
3. SARS-CoV-2 VARIANTS
SARS-CoV-2 has adaptive mutations like all viruses in the viral genome that can accumulate and change its genetic code, modulating the SARS-CoV-2 pathogenic potential. SARS-CoV-2 is known to have an intrinsic repair mechanism and, because of this, mutations have occurred at a slower rate than other RNA viruses. On average, the genome obtained in October 2020 from SARS-CoV-2 had 20 mutations in its spike protein compared to the genome of wildtype SARS-CoV-2 obtained in January 2020 [19]. SARS-CoV-2 is evolving at a rate of 1×10-3 amino acid substitutions per year, where one substitution occurs every 11 days [18]. Based on transmissibility rate, ability to evade host defense mechanism, decrease the neutralization of infection by host antibodies, and the widespread impact on public health, a few variants are considered to be variants of concern (VOCs).
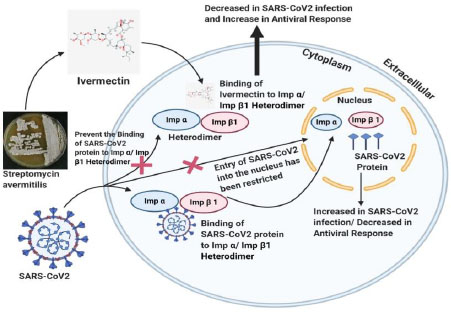
3.1. Variants of Interest
A variety of unique genetic markers that are associated with changes in receptor binding, reduced neutralization with previously generated antibodies. They are also correlated with reduced therapy effectiveness, possible diagnostic impacts, or expected increased transmissibility. Variants of concern might require suitable measures for public health, e.g., enhanced sequence surveillance, enhanced laboratory characterization, or epidemiological surveys to determine the ease with which the virus is transmitted to others. The seriousness of the disease, therapeutic effectiveness, and whether vaccines currently approved are protective are major concerns of public health [17, 19]. The epidemiological update by WHO, as of 31-May 2021, described seven variants of interest (VOIs). These VOIs are B.1.427/B.1.429, P.2, B.1.525, P.3, B.1.526, B.1.617.1 and, C.37.The current variants of interest which are circulating worldwide are shown in Table 1 and Fig. (2).
Variant Name Based on Pango Lineage (WHO Label) | Notable Mutations | GISAID Lineage | First Reported | Attributes |
---|---|---|---|---|
B.1.427/B.1.429 (Epsilon) | L452R, D614G,/ S13I, W152C, L452R, D614G | GH/425R.V1 | California, the USA in July 2020 | 1. 20% more infectious 2. Promotes evasion from host defenses and reduces monoclonal antibodies neutralization |
P.2 (Zeta) | E484K, D614G F565L*, V1176F |
GR/484K.V2 | Brazil in April 2020 | 1. Promotes evasion from host defenses and reduces monoclonal antibodies neutralization |
B.1.526.1/ B.1.526 (Iota) | D80G, L452R D950H, F157S D614G T791I* Δ144,T859N*, S477N*,L5F* D253G,E484K* |
GH/253G.V1 | New York in October/ November 2020 | 1. Promotes evasion from host defenses and reduces monoclonal antibodies neutralization 2. Reduces shedding of S1 of spike protein and enhances its infectivity rate |
B.1.525 (Eta) | A67V, F888L Δ144, E484K Δ69/70, D614G Q677H |
G/484K.V3 | Multiple countries in December 2020 | 1. Promotes evasion from host defences and reduces monoclonal antibodies neutralization |
P.3 (Theta) | E484K, N501Y, P681H, 141-143del | GR/1092K.V1 | The Philippines in January 2021 | 1. Promotes evasion from host defenses and reduces monoclonal antibodies neutralization |
B.1.617.1 (Kappa) | G142D, E154K, L452R, E484Q, D614G, P681R, Q1071H | G/452R.V3 | India in October 2020 | 1. Promotes evasion from host defenses and reduces monoclonal antibodies neutralization |
C.37 (Lambda) | L452Q, F490S, D614G | GR/452Q.V1 | Peru in August 2020 | Unclear |
3.2. Variants of Concern
Variant shows an increase in transmissibility, more severe disease (e.g., increasing hospitalizations or death), a substantial decrease in antibody neutrality produced during pre-infection or vaccination, decreased efficacy or failure to identify diagnoses, etc. Any variant of concern might require appropriate public health action, such as notification of vaccine efficacy to the WHO under the International Health Regulations, reporting to CDC, local or regional efforts to control spreading, etc. Additional factors may also include in order to create new diagnostics measures or modify vaccinations or therapies based on the characteristics of this variant [19]. The first variant of concern, the B.1.1.7 (Alpha), was obtained in the United Kingdom on 18-December 2020, followed by B.1.351 (Beta) in South Africa. On 11-January 2021, the P.1 (Gamma) variant of concern was reported in Brazil. More recently, the new variant B.1.617.2 (Delta) was reported in India in early October 2020 and is considered VOC on 11-May 2021. All four said variants of concern (Alpha variant, Beta variant, Gamma variant, and delta variant) have amino acid substitution in the N-terminal domain (NTD) and the receptor-binding domain (RBD), whereas N501Y mutation was common in all the variants. The current variant of concern that is circulating worldwide is listed in (Table 2 and Fig. 1)
4. NEED FOR IMMUNOMODULATORS
The pathogenesis of COVID-19 caused by SARS-CoV-2 has not been understood thoroughly. It has been assumed that the host's immune response plays a significant role and it was found that abnormal immune response causes lung tissue damage, respiratory system failure, and reduced lung capacity [20]. Dendrite cells and macrophages produce cytokines, and chemokines, like IL-12, IL-6, IFN-γ, and TNF-α, and granulocyte-macrophage colony-stimulating factors, stimulate inflammation [6]. The cytokine storm is an excess of cytokines triggered by an abnormal immune response. Cytokine storm is a vital factor in disease progression and ultimate death in the later stage of coronaviruses, like SARS, MERS, and COVID-19 [21]. The pro-inflammatory Th-17 cells, perforin, and granulysin produce cytotoxic T cells, which increase significantly. They are responsible for causing severe immune injury within the lungs of the COVID-19 patients [22, 23].
Any biological/non-biological agent that alters the host's immunoregulatory response is called immunomodulatory. They could be immune-suppressor, immune-stimulator, and even referred to as immunomodulators. Cytokines and chemokines regulate immune responses; however, viruses can evade or even mimic their signaling pathway. For the physiological stability of an organism, immunomodulation becomes very important. To reduce these immunomodulators' side effects, it becomes vital to search for alternative natural sources [24]. IL-1 and JAK inhibitors (baricitinib) are an example of specific immunomodulators. Whereas, corticosteroids, like dexamethasone, interferons, and hydroxychloroquine, are related to non-specific immunomodulators [25].
4.1. Vitamins and Micronutrients as Immune Boosters
Ascorbic acid, commonly termed vitamin C, is a water-soluble vitamin that humans and other primates cannot synthesize. Vitamin C, due to its multiple roles in many metabolic pathways, contributes to immune-modulating effects. It is a potent antioxidant because it reduces damaging ROS. It can forage many reactive oxidants species, like superoxide radicals, peroxyl radicals (aqueous), etc. It can restore the active forms of the essential membrane antioxidants, including glutathione and vitamin E (tocopherols), hence acts like a co-antioxidant [26, 27]. It has been observed that high ascorbate levels have stimulatory effects on neutrophil mobility. The mechanism is linked with the inhibition of the MPO/H2O2/Halide system, preventing the neutrophil membrane's auto-oxidation. Studies have suggested that ascorbic acid results in an enormous proliferation of natural killer cells. Due to its immune-modulating functions, it was tested in patients and showed positive results since it reduced mortality and symptoms [28].
Variant Name Based on Pango Lineage (WHO Label) | Notable Mutations | Number of Mutation [Mutation Number in Spike] | First Reported | Attributes |
---|---|---|---|---|
B.1.1.7(Alpha) | N501Y, 69–70del, P681H | 23 [8] | United kingdom in October 2020 | 1. High transmissibility rate (50% higher) 2. High infectivity rate compared to other non-VOC lineages 3. Enhanced reproductive rate |
B.1.351 (Beta) | N501Y, K417N, E484K | 21 [9] | South Africa in December 2020 | 1. 50% high transmissibility rate 2. Resistance to antibodies effect |
P.1 (Gamma) | N501Y, E484K, K417N | 17 [10] | South Africa in December 2020 | 1. Maybe resistant to vaccines 2. Promotes evasion from host defenses and reduces monoclonal antibodies neutralization |
B.1.617.2 (Delta) | L452R, T478K, D614G, P681R | 12 [9] | India in December 2020 | 1. Promotes evasion from host defenses and reduces monoclonal antibodies neutralization 2. High transmissibility rate |
Vitamin D, which plays various roles in the body, acts on both innate and adaptive immunological responses. Vitamin D boosts the innate immune system by upregulation of antimicrobial peptides, including cathelicidin and defensins [29]. While vitamin A influences epithelial integrity, it plays a part in developing the epithelium as a barrier function; it also regulates the differentiation, maturation, and activity of macrophages and neutrophils to boost killing and phagocytosis [30]. The research indicates that zinc, vitamin D, and calcium are essential components of the immune system and perform synergistic functions at different phases of host defenses, like preserving the integrity of biological barriers and the functionality of cells that comprise the innate and adaptive systems [31]. Vitamin B6 and B12 directly impact leucocytes and CD4+ T cells. These cells play a critical role in establishing an adaptive immune response to a wide range of pathogens. Furthermore, folic acid promotes T-cell proliferation [32]. Each of these vitamin and mineral intakes might enable recovery from COVID-19 infection. Yet, there is still a scarcity of preclinical and clinical research on vitamins and minerals in the treatment of COVID-19 [33]. Large doses of all these nutrients demonstrated beneficial outcomes during COVID-19 infection, and due to their low level of risk, these are an excellent supplement to patient care [29].
4.2. Anti-inflammatory and Immunosuppressive Drugs
4.2.1. Dexamethasone
An inexpensive and commonly used steroid for treating patients with severe SARS-CoV-2 infection has been observed to save lives in a randomized controlled clinical investigation in the United Kingdom. Clinical trials indicated that dexamethasone usage reduced deaths in COVID-19 patients by one-third who were on ventilators, and improvement was seen in patients receiving oxygen therapy but were not on a ventilator. However, it did not affect the patients who were not severely ill [34]. Dexamethasone and other exogenous corticosteroids have a similar mechanism of action. CTLA-4mRNA upregulated, but CD-28 mediated cell cycle entry and differentiation was blocked by dexamethasone. Dexamethasone reduces differentiation and naïve T-cell proliferation by weakening the CD-28 co-stimulatory pathway. In ARDS, to restore hemostasis, pulmonary and systemic inflammation should be downregulated [35, 36]. Protracted glucocorticoid therapy has been linked to significant improvements in alveolar-capillary membrane permeability, as well as inflammation and tissue repair mediators. As per the Randomized Evaluation of COVID-19 Therapy (RECOVERY) study, dexamethasone lowers death in COVID-19 hospitalized patients. Dexamethasone's positive impact was most noticeable in individuals who were on mechanical ventilation [37]. The RECOVERY research reveals that treating patients of COVID-19 who required respiratory assistance with dexamethasone at a dose of 6 mg once every day for up to 10 days lowered 28-day mortality. However, no advantage was observed in those who did not require oxygen [38]. Overall, the results reinforce the hypothesis that early dexamethasone therapy may alter systemic immune responses while also shortening the duration of mechanical ventilation and lowering the mortality rates in general [39].
4.2.2. Tocilizumab
Tocilizumab, the first marketed IL-6 inhibiting monoclonal antibody that targets IL-6 receptors, has demonstrated safety and efficacy in treating rheumatoid arthritis [40]. However, in one open-label, randomized and controlled trial involving relatively young patients hospitalized with verified serious COVID-19, the interleukin-six inhibitor tocilizumab did not lead to improved clinical outcomes as measured by a seven-level ordinal scale after 15 days [41]. Conversely, in the survey of 3924 critically ill COVID-19 patients admitted to ICUs at 68 hospitals all across the United States, during the first two days of ICU admission, the patients who received tocilizumab had a lower risk of death compared to those who did not receive tocilizumab during the first two days of ICU admission [37]. Tocilizumab, an IL-6 inhibitor, unquestionably plays a significant role in reducing cytokine storms that can considerably alleviate the clinical symptoms.
Furthermore, no treatment-related adverse responses or subsequent lung infection were documented with tocilizumab use [42]. Recent findings have shown that tocilizumab treatment successfully reduces mortality in severe COVID-19 patients. It regulates excessive inflammation and boosts immunological function. However, these conclusions must be verified through well-designed randomized controlled trials [43].
4.2.3. Hydroxychloroquine
Hydroxychloroquine, an anti-rheumatic and antimalarial drug, demonstrates immunomodulatory effects but not immunosuppression. It is widely used in systemic lupus erythematosus or as an antiviral agent against viral infections, like SARS, influenza, etc [44]. Studies have also reported that hydroxychloroquine has antiviral characteristics and has efficacy in treating COVID-19, as does chloroquine. For viruses, cell entry and replication depend on their internalization through endocytosis (Fig. 3). The pH-dependent fusion of viral outer membrane with lysosomal/endosomal membrane releases nucleocapsid content [45]. Hydroxychloroquine is a weak base. It raises the pH of the lysosomal/ endosomal compartments and thus interferes with viral fusion. Reports have also shown that hydroxychloroquine hinders the pathway by which processed epitopes are displayed by antigen-presenting cells and affects autophagy [46, 47]. It also inhibits terminal glycosylation of the ACE2 receptor that reduces the efficiency of binding between the host cells ACE2 and the spike protein of SARS-CoV/ SARS-CoV-2 (Fig. 4) [48, 49]. Hydroxychloroquine is an anti-inflammatory agent that is safe and used against autoimmune diseases. Also, it significantly decreases cytokine production by T cells and dendritic cells (e.g., IL-6, IL-1, and TNF α), supporting the hypothesis that it can suppress chronic respiratory syndrome triggered by over-activation immune system due to SARS-CoV-2 infection. Hence, it contributes to attenuating the inflammatory response in patients with coronavirus, and the study also showed that it can effectively inhibit SARS-CoV-2 in vitro [50]. When results from RCTs were pooled, HCQ use was not found to be linked with death in COVID-19 patients. However, it was found to be related to a 20% mortality reduction after merging the data from observational studies (low level of certainty of the evidence). The decrease in fatality was most noticeable in observational studies that utilized lower dosages of HCQ [19]. However, in one observational study that assessed individuals hospitalized for COVID-19 treated with HCQ/AZI combination therapy with a 28-day follow-up, no improvement in survival without mechanical ventilation was seen compared to those who were not [51]. It is also associated with side effects, as warned by the FDA (Food and drug administration, US). It is reportedly related to cardiotoxic events such as QT prolongation and ventricular arrhythmias [52]. A separate study on a sizeable Iranian group tends to support early use (during the first 3–7 days after COVID-19 detection) of HCQ in moderate COVID-19 infection in an outpatient setting for lowering hospitalizations and mortality with no significant adverse HCQ-related effects. Clinical trial results must validate these observations because HCQ is a cheap and widely available medicine that could still contribute to decreasing the COVID-19 burden in a particular population, notably in resource-poor nations [53].
5. REPURPOSED ANTIVIRAL DRUGS
5.1. Remdesivir
Remdesivir (GS-5734) is an experimental nucleoside analog approved by US-FDA (Food and drug administration, USA). It is a broad-spectrum antiviral monophosphoramidate prodrug which is an adenine derivative formulated by Gilead Sciences [54]. Remdesivir (RDV) has the potential to inhibit the replication of coronavirus pathogens, like MERS-CoV and SARS-CoV [54]. Both these coronaviruses are structurally similar to SARS-CoV-2, which led to the usage of RDV in treatment [55, 56]. RNA Dependent RNA Polymerase (RdRp) is a major enzyme involved in genomic replication. RdRp is usually found conserve in the members of viruses in a family, making it an ideal target for antiviral therapy. Once the virus invades the host cell, it uses its viral genomic RNA as a template for the synthesis of RdRp, which is required for protein synthesis of the host cell. RdRp further completes transcription of sub-genomic RNA, viral genomic RNA, and structural protein-related mRNA [57] (Fig. 2). Such a key role of the polymerase makes it an ideal target for broad-spectrum antiviral drugs. RDV enters the host cell in the form of a prodrug and gets converted to RDV-Monophosphate. Its active form, RDV-triphosphate, is similar to ATP (adenosine triphosphate) and competitively binds to the RdRp of viruses with similar efficacy. Under the US FDA’s compassionate use guidelines, this drug was initially allowed to be administered in a US patient and showed successful treatment [58]. Using the data obtained from ACTT (Adaptive COVID-19 Treatment Trial), EUA (Emergency Use Authorization) has been given to Remdesivir [59]. Currently, 200 mg of Remdesivir can be given intravenously on day 1, followed by 100 mg daily for nine days. RDV can decrease viral load in the lungs and alleviate clinical symptoms and also respiratory function. These findings support the use of this medication to treat severe human coronavirus infections [60]. RDV outperformed other antivirals in reducing SARS-CoV-2 infection (EC50 = 0.77 M) such as ribavirin (EC50 = 109.5 M), penciclovir (EC50 = 95.96 M), nitazoxanide (EC50 = 2.12 M), chloroquine (EC50 = 1.13 M), hydroxychloroquine (EC50 = 0.77 M), and favipiravir (EC50 = 61.9 M) [61].
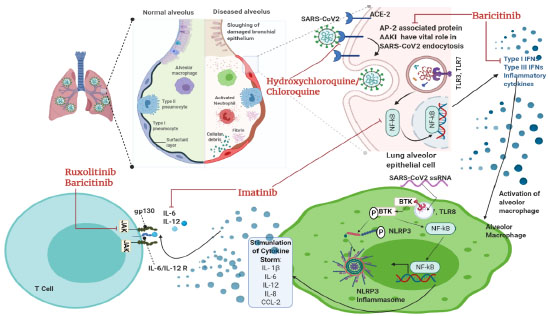
A few of the side effects of the drugs are diarrhea, abnormal liver function, rashes, low blood pressure, nausea, and vomiting [56]. The authors suggested that studies on the use of RDV in the therapies of COVID-19 are constrained by the limited size of the group, the short period of follow-up, possibly incomplete data given the nature of the program, a lack of details on patients treated at baseline, and the lack of a randomized control group [62-63]. A meta-analysis comprising three randomized controlled trials with a total of 1691 patients showed that remdesivir enhanced the probabilities of hospital release; however, remdesivir therapy did not lower the likelihood of death in COVID-19 patients. The findings show that remdesivir is beneficial at slowing illness development and hastening patient recovery, especially when given early in the illness stage. However, on the other hand, it may be less beneficial in improving recovery periods in severely sick patients [62].
5.2. Favipiravir
Toyama Chemical Co., Ltd. developed favipiravir by exploring a chemical library for antiviral activity towards the influenza virus [64]. Favipiravir is a pyrazine carboxamide derivative that selectively inhibits the virus's RdRp and prevents replication (Fig. 2). The phosphoribosylated form of favipiravir, favipiravir-RTP (favipiravir-ribofuranosyl-50-triphosphate), inhibits the RNA polymerase enzyme. HGPRT (human hypoxanthine-guanine phosphoribosyl-transferase) enzyme has been observed to play a vital role in converting favipiravir to its active phosphoribosylated form [65]. The catalytic domain of RNA polymerase recognizes this dynamic form of favipiravir and favipiravir blocks its catalytic activity.
No toxic effect of this drug is seen in mammalian cells and it does not affect their nucleic acid synthesis [65]. This drug is completed its phase-III clinical trial in Japan and is undergoing a phase-III clinical trial in the USA. In March 2020, Favipiravir was approved for the treatment of COVID-19 patients in China [56]. According to Fujii and colleagues, favipiravir therapy is related to the early reduction of fever in COVID-19. The timely start of favipiravir medication may reduce the length of COVID-19 treatment [66]. Udwadia et al. concluded that early oral favipiravir treatment might shorten the length of clinical manifestations in people with moderate COVID-19, as seen by a considerably shorter time of clinical cure [67-68]. Consistent with the previous findings, Hassanipour et al. found in their meta-analysis a drop in all-cause fatality in individuals who took Favipiravir compared to those who received control medications. They also noted that Favipiravir treatment had minimal acceptable side effects such as nausea, vomiting, diarrhea, and increased serum transaminases [67]. However, several studies have demonstrated that favipiravir exposures decline over time, rendering dosage difficult [69].
6. KINASE INHIBITORS AS A TREATMENT OPTION
6.1. Ruxolitinib
Ruxolitinib, also known as Rux or by its trade name Jakavi, is a highly effective and potent selective inhibitor of Janus Kinase (JAK) 1 and 2. It also inhibits the action of JAK-3 and Tyrosine Kinase-2 with comparatively lower potency [70] (Fig. 4). Approved by the European Union (EU), this drug is utilized in the treatment of diseases like PV (Post-Polycythemia Vera) and PMF (Primary Myelofibrosis) [71]. Rux shows high efficacy due to its broad anti-inflammatory properties against MPN (Myeloproliferative neoplasm), which plays a crucial role in causing cytokine storm due to inflammation-causing cytokines and interleukins [72]. In COVID-19, severely affected patients develop ARDS (acute respiratory distress syndrome), which ultimately causes multiple organ failures linked with the release of pro-inflammatory cytokines. The condition is addressed as a cytokine storm. Upon administering a cumulative dosage of Rux of 135mg per day for nine days, a reduction was seen in CIS score in COVID-19 patients. The motive was to administer Rux for a short-term duration which could help balance the cytokine storm without completely suppressing the immune system, which may cause reactivation of the virus. The end goal of this treatment is to stop or prevent further damage to the organs in severely affected COVID-19 patients. A pilot study concluded that in an initial group of patients impacted by COVID-19, though not in extreme respiratory distress needing invasive ventilation, the use of Rux as a compassionate treatment reduces the hyper inflammatory state, leading to more efficient ventilation and faster recovery [73].
6.2. Imatinib
Imatinib, a tyrosine kinase inhibitor drug, is a US-FDA-approved [74]. It is used to treat a tumor in CML (chronic myelogenous leukemia) and gastrointestinal stromal tumors. This drug functions by targeting BCR-ABL fusion protein [75]. It also inhibits C-KIT, platelet-derived growth factor receptor, and ABL-1/2. Imatinib has immunomodulatory properties and attenuates pro-inflammatory molecules, like IL-6, NF-kB, and TNF-alpha, which are key molecules involved in the progression of cytokine storms. The primary mechanism of action for imatinib can be seen in Fig. (4). It reduces pulmonary edema, prevents any histological damage, and improves endothelial barrier dysfunction [76]. Due to these characteristics, imatinib was considered as a potential drug that could help alleviate ARDS and prevent multiple organ failure in severely affected COVID-19 patients [77, 78]. It has also shown antiviral activity against MERS and is expected to treat SARS-CoV-2. In SARS-CoV-2, the virus is thought to undergo cell fusion with the help of ABL-2. Imatinib functions by blocking ABL-2 and prevents the spread of infection. This treatment is believed to be very effective when administered early in the stages of the disease. A recent trial published in The Lancet Respiratory Medicine found that imatinib does not shorten the time to cessation of supplementary oxygen and mechanical ventilation in hospitalized COVID-19 patients. However, the decline in fatality suggests that imatinib may provide a therapeutic effect in individuals with COVID-19, justifying additional research [79]. WHO will put three known drugs to the test, one of which is imatinib. It may tackle both the coronavirus and inflammation, prevent viral penetration into human cells, and decrease the release of pro-inflammatory molecules known as cytokines [80].
6.3. Baricitinib
Baricitinib is an orally taken JAK inhibitor that is JAK1 and JAK2 specific [81]. It is reported to suppress the cytokine storm due to high cytokine release. Hence, it can be regarded as an anti-inflammatory. Initially, this drug was licensed to treat RA (rheumatoid arthritis). The antiviral effect of baricitinib is observed due to its affinity to AP-2 associated protein-AAKI. AAKI plays a significant role in SARS-CoV-2 endocytosis, inhibited by baricitinib [82] (Fig. 4). A trial with a limited number of patients was conducted, which was funded by Azienda-USL, Toscana Centro Committee for off-label drug use. Total 4mg of baricitinib/day was orally administered for 14 days along with Lopinavir/Ritonavir. No severe AE was observed in the patients after baricitinib treatment. No indications of bacterial, opportunistic infection, trombone-felitis, or hematologic toxicity were observed. In the case of COVID-19 disease, baricitinib has been identified as a promising anti-inflammatory drug. According to a recent study in Bangladesh, 8 mg daily oral intake of baricitinib for 14 days resulted in earlier stabilization of respiratory function, less need for ICU and intubation assistance, a lower 30-day death rate, and a lower 60-day re-hospitalization level compared to a dose of 4 mg/day [83]. When corticosteroids are not an option, the National Institutes of Health (NIH) panel advises using baricitinib in conjunction with remdesivir for treating COVID-19 patients that are hospitalized, non-intubated, and need oxygen support. The panel's revised guidelines for using baricitinib based on COV-BARRIER results, a multinational, randomized, placebo-controlled trial [81].Overall, the findings associated with an in vitro study results show that baricitinib acts when a significant cytokine release occurs, implying that therapeutically, this treatment may be beneficial before the development of an aggravated immune response. Baricitinib-treated COVID-19 patients consistently had lower pro-inflammatory cytokines (IL-6, TNF-, and IL-1) than baseline [84].
7. ANTIPARASITIC DRUGS/ ANTIBIOTICS AS A POSSIBLE THERAPEUTICS
7.1. Ivermectin
Ivermectin is a US FDA-approved broad-spectrum anti-parasitic drug. In vitro experiments with ivermectin have shown antiviral activity against a wide range of viruses [85]. Initially, ivermectin was reported to inhibit the import of host and viral proteins across the nuclear membrane [86]. It inhibits the interaction between human immunodeficiency virus I (HIV-1) and importin (IMP) α/β1 heterodimer. It is responsible for the nuclear import of integrase protein, as shown in Fig. (3). Later on, its ability to stop the replication of HIV was confirmed. In terms of SARS-CoV-2 infection, ivermectin is expected to target IMP α/β1 heterodimer by destabilizing it. It also prevents its binding to the viral protein, inhibits the entry of the viral protein into the nucleus, and lowers its ability to shut down antiviral response.
Critically ill SARS-CoV-2 patients are recommended a 150 μg/Kg dosage, which showed reduced mortality rate and resource requirement in the treatment. Few pharmacokinetics problems associated with ivermectin are cytotoxicity and low solubility. To overcome this, many liposomal systems and ivermectin nano-carriers are used in cell lines. They lowered cytotoxicity concerning the free ivermectin molecule [87]. The golden hamster model findings showed that IVM exerts anti-inflammatory activity in trial COVID-19 infection [88]. Preliminary research has found a propensity to reduce viral loads in the ivermectin cohort, as well as an ability to limit IgG titers, which may represent milder illness and clinical improvement in COVID-19 core symptoms linked with tissue injuries such as anosmia/hyposmia and coughing. These findings are consistent with evidence from studies in other countries that indicate quicker viral clearance in treated subjects [89]. However, the medicinal safety of IVM usage in kids, the aged, and pregnant women is currently relatively restricted. Further studies are urgently needed to optimize dose according to patient condition, age group, and risk assessment, particularly considering ivermectin's propensity for neurological, hepatotoxicity, and drug resistance/tolerance [90].
7.2. Azithromycin
Azithromycin, a macrolide antibiotic, earlier exhibited promising effects on patients of COVID-19. Its anti-inflammatory, antiviral, immunomodulatory, and antimalarial characteristics made it especially well-suited not only to prevent this novel, highly contagious virus but also to alleviate the potentially fatal symptoms associated with its infection [91]. However, according to the Randomized Evaluation of COVID-19 Therapy (RECOVERY) study, azithromycin cannot be considered an appropriate treatment for COVID-19 hospitalized patients. Referral of azithromycin is not linked to a decline in deaths and length of hospital stay [92]. Sivapalan et al. reported that the combination of azithromycin and hydroxychloroquine did not improve the chances of survival [93]. Data from the UK-based, randomized trial (PRINCIPLE) indicate that, even after probable antiviral and anti-inflammatory characteristics, azithromycin is ineffective in treating COVID-19, even though it was previously used during the disease [94].
8. CONVALESCENT PLASMA TRANSFUSION (CPT)-A DUBIOUS HOPE
CPT is another hopeful treatment option that has emerged recently. The Convalescent Plasma (CP) is collected using an apheresis tool from recovered individuals. In terms of SARS-CoV-2 infection, antibodies have been developed against the pathogen and then transfused into another infected patient having a severe response to the virus, as shown in Fig. (5). It is a passive immunization strategy in which antibodies derived from CP can subdue a virus by inhibiting replication or binding without interfering with replication. Its administration after symptom onset showed reduced mortality rates compared to their placebo group in severe acute respiratory infection of viral etiology like SARS-CoV [95, 96]. Neutralizing antibodies (NAbs) obtained through CP restrain the infection, and its efficacy is linked with the NAbs concentration in the plasma from the recovered patient [97]. Following the FDA's (emergency use authorization) EUA in late August 2020, the usage of CCP in hospitalized patients increased significantly.
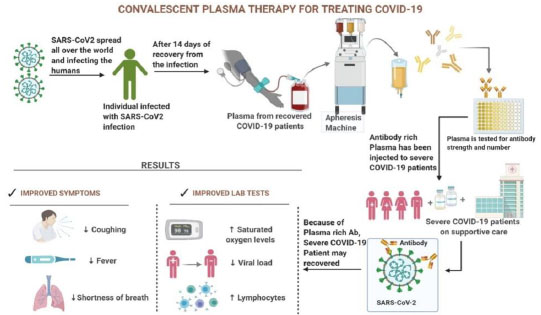
Conversely, RCTs from India, Argentina, and the United Kingdom found that CCP usage did not decrease mortality in hospitalized patients. This claim was accompanied by a significant drop in its use that started in November 2020 [98]. As per a new scientific report, the following variables can be contributed to the failure of CCP treatment in treating COVID-19 patients in India:
1) Using CCP lacking high titer neutralizing antibodies for SARS-CoV-2 due to lack of testing, usage of such CCP will be futile [99],
2) It was shown that using polyclonal CCP to treat COVID-19 in an immunocompromised patient led to developing a viral mutant strain with immunological escape potential [100].
COVID-19 is a health emergency across the globe with still no definitive drug or vaccine to treat it; therefore, CPT may be considered due to the overall low incidence of worsening of the condition and since it has been observed to reduce virus load if given early in treatment in critical patients [101]. Also, because it is easily accessible to patients, does not need complicated production, and is cheap [102]. Transfused COVID-19 patients had a considerably lower fatality rate compared to non-transfused COVID-19 patients. Further research found that timely infusion of high-titer plasma decreases death in COVID-19 patients. These findings support the use of convalescent plasma as a COVID-19 treatment agent [103].
With a scarcity of effective anti-SARS-CoV-2 treatments, there is an urgent need for further randomized and controlled plasma therapy-based research trials on COVID-19 patient outcomes. As the convalescent plasma therapy becomes a ray of hope in COVID-19 management, it is still far from being clarified definitively [104].
9. ANTIBODY COCKTAIL
Neutralizing antibodies or NAbs are being used as a SARS-CoV-2 treatment option. These exogenous antibodies generally identify regions in RBD (Receptor Binding Domain) and in some cases, NTD (N-Terminal Domain) of the spike protein as an epitope. More than 20 NAbs are undergoing various clinical trials, and a few moved on to the 2nd or 3rd phases of their trial. These are being evaluated for the treatment of ambulatory and hospitalized COVID-19 patients. Detailed efficacy data is only available for a cocktail of bamlanivimab/ etsemivab developed by Eli Lilly & AbCellera (NCT04427501) and a cocktail of casirivimab (REGN 10933) and imdevimab (REGN 10987) acquired by Regeneron & F. Hoffman- La Roche ltd. (NCT 04425629). Emergency use authorization has been given to both the NAb cocktails [105]. RegeneronNAb cocktail, also known as REGEN-COV, which uses two potent virus-neutralizing antibodies, binds non-competitively to the RBD of SARS-CoV-2 spike protein. This treatment showed reduced escape of mutant viruses and imparted protection against variants of spike proteins. US-FDA and CDSCO India issued REGN-COV EUA [106]. In India, it will be produced by CIPLA for pan-India to treat mild-to-moderate COVID-19 cases in adults and pediatric patients of >12 years with a minimum weight of 40 kgs. This treatment is only to be used for those with a confirmed case of SARS-CoV-2 and is in its initial stages with a risk of progression to the severe condition due to co-morbidities mentioned in the ‘Fact Sheet for Healthcare Providers.’ Patients must be in the initial stages without the requirement for supplemental oxygen. The currently available dosage is 2400mg via intravenous infusion [107].
10. VACCINE- BOTH A PROPHYLACTIC AND A THERAPEUTIC
COVID-19 vaccines are being hailed as the quick fix for offering the most potentially efficient and productive way of putting the contagion to rest, notably considering the scarcity of targeted therapies for the SARS-CoV-2 virus [108]. A vaccine must induce the production of selective and neutralizing antibodies. The objective is to introduce the person to an antigen that increases the immunological response, inhibiting or eradicating the virus in the event of infection while avoiding the stimulation of COVID-19 [109]. The anti-SARS-CoV-2 proposed vaccines can target either the entire SARS-CoV-2 or the components or parts of molecules expressed on the virus surface, using various technologies. These different candidate vaccines could be classified according to the technology platform used to induce a protective immune response [110]. According to a WHO report (https://www.who.int/publications/m/item/draft-landscape-of-COVID-19-candidate-vaccines), there are presently 287 potential vaccines in development, from which 102 are in clinical trials, comprising 32 protein subunit vaccines, 10 DNA vaccines, 16 RNA vaccines, 16 viral vector vaccines, as well as others.
Several vaccine candidates have gained emergency use approvals so far, and several nations have begun vaccination implementation initiatives [111]. A comparative analysis of different vaccine platforms is summarized in Table 3.
Here, different types of vaccines are summarized as follows:
10.1. Whole Virus Vaccine
One type of vaccine is the whole virus vaccine that employs inactivated full virus or lives attenuated virus, representing a conventional strategy for viral vaccinations [112]. This platform is preferable due to its inherent immunogenicity and capacity to stimulate Toll-like receptors; however, safety concerns are still present in this type of vaccination strategy due to inefficient inactivation or attenuation of the viruses. Coronavirus vaccines are mainly an issue. Furthermore, an increased infectivity rate after immunization with this type of vaccine is observed in some findings [113].
10.1.1. Strategy for Live Attenuated SARS-CoV-2 Vaccine
Live attenuated-based vaccines may be obtained by culturing SARS-CoV-2 in an unfavorable environment or making a genetically weakened virus. However, attenuation of the virus is a complex process and may be associated with biosafety risks. The live attenuated vaccines demonstrated few side effects like attenuated viruses can cause diseases, even if it is administered in small quantities.
10.1.1.1. Only three live attenuated-based vaccines are in pre-clinical development:
- COVI-VAC (Intranasal live attenuated vaccine) was developed by the Serum Institute of India in collaboration with Codagenix, New York.
- Griffith University, Australia, in collaboration with the Indian Immunologicals Ltd., India
- Mehmet Ali Aydunar University, Turkey
All these vaccines are in pre-clinical phases and have not yet reached the clinical development phase.
10.1.2. Strategy for Inactivated SARS-CoV-2 Vaccine
The vaccines produced by inactivating the SARS-CoV-2 virus are more stable than vaccines produced by genetically weaken SARS-CoV-2. The immune memory is developed for a short duration, demanding the administration of a higher quantity of vaccine or administration of inactivated virus with the carrier. The induced response by inactivated virus vaccines is usually weaker than the response by attenuated virus vaccines.
Seven inactivated virus-based vaccines are in clinical trials. Out of seven, four vaccines have entered phase III clinical trials and are approved for emergency (limited) use. Phase 2 clinical data shows that the inactivated virus vaccine is safe and induces high antibody titer.
10.1.2.1. The seven inactivated virus vaccines are:
• Coronavac by Sinovac Biotech showed positive results in the 1 and 2 phases of clinical trials and has now entered the third phase with 92% efficiency.
• Covaxin by Bharat Biotech has completed its phase III clinical trial and showed 81% efficiency.
• Sinopharm/BIBP vaccine was approved for emergency use and showed 79% efficiency at phase III clinical trials.
• Sinopharm/WIBP vaccine was approved for emergency use in general populations and showed 72% efficiency in phase III clinical trials.
• IMBCAMS, China, is still under development.
• QazVac was developed by RIBSP, Kazakhstan, and approved for emergency use only in Kazakhstan.
10.2. Subunit Vaccines
This platform is considered safe, effective, and potent because it does not include whole virulent virus but consists only of antigens that might be engineered peptides or proteins, expressing only a part of the immunogen. Many structural proteins, including spike (S), envelope (E), and others, can generate immune response since they can generate NAbs by acting as an antigen, expressed by SARS-CoV-2.
10.2.1. Strategy for Subunit SARS-CoV-2 Vaccine
For SARS coronavirus 2, the subunit vaccine relies on elevating an immune response against the S protein, which prevents its interaction with the host ACE2 receptor [113]. These vaccines contain adjuvants, which could be of synthetic or bacterial origin, to stimulate robust host defense response.
Table 3.
Vaccine strategies | Example | Side effects | Advantages | Disadvantages | Efficiency | Clinical Trial Phases and Reference |
---|---|---|---|---|---|---|
DNA | INO-4800 | • Nausea • Headache • Pain in muscle • Reaction at the injection site |
• Handling of infectious material is not needed • Reasonable and stable • High-speed manufacturing • Easy to design and manipulate |
• Has a risk for host cell genomic integration • variable and low immune response, • No approved DNA vaccine |
1mg dose group demonstrates 78% neutralizing antibodies whereas 2 mg dose group shows 84% neutralizing antibodies | Undergoing Phase II/III [137, 138] |
RNA | 1. mRNA-1273 2. BNT162b mRNA |
• Weakness • Headache • Swelling at the injection site. • Myalgia • Fever • Rashes and itching • Quality of sleep degrades • Fatigue • Feeling of lethargy • Mild to moderate pain in hand. • Joint pain |
• Handling of infectious material is not needed • Strong antiviral responses • Insertional mutagenesis not seen • High-speed manufacturing • Depicts enhanced immunogenicity • Ensures efficient performance • They develop rapidly • Pure viral protein is produced without the risk of integration with the host genome • Rapid commercial production |
• May induce inflammatory responses. • No FDA approved RNA vaccine • Boosting is necessary • Systemic and local adverse effects were produced. • Allergic reactions were produced. • Less stable. |
94.1% 95% |
Completed Phase III Clinical trial [139, 140] Completed Phase III Clinical trial [117] |
Protein | RBD-Dimer (ZF2001) | • Body aches • Fatigue • Nausea |
• Strong antibody response • Successful platform • Large scale production capacity |
• Adjuvants needed • Multiple boosters are required. |
Completed Phase III clinical trial | |
Viral Vector (Replicating/ non-replicating | 1. AZD-1222 (Covishield) 2. GRAd-CoV2 3. Ad5-Ncov (Convidecia) 4. Sputnik V (Gam-COVID-Vac) 5. Ad26COVs1 |
• Reduced energy • Malaise (sensation of discomfort) • Muscle pain • Headache • Vomiting • Tiredness • Joint pain • Swelling in arms • No such side effects • Mild headache • Fatigue • Weakness • Mild pain in the arm • Short-lived allergies • Redness at the injection site • Mild fever • Muscle pain • Feeling of heaviness in the body |
• Immune induction is excellent • High-level protein expression • Long term stability • Single-dose induces long-term protection. • Boost cellular immunity • The delivery of antigen is precise • Contact with any infectious particle is avoided |
• May induce inflammatory responses • Immune response to vector is generated. • It can cause cancer since the viral genome has the potential to get incorporated into the host. |
70.4% effective against B.1.1.7 81.5% effective against non-B. 1.1.7 lineage 65.28% effective in preventing all symptoms in patients 28 days after 1st dose and shows 90.7% effectiveness in preventing severe COVID-19, 28 days after 1st dose 91.6% effective against confirmed COVID-19,21 days after 1st doses 85% effective in preventing severe COVID-19, 28 days after 1st dose |
Completed Phase III clinical trial [141, 142] Entered Phase II/III Clinical trial Completed Phase III clinical trial [143] Completed Phase III clinical trial [144, 145] Completed Phase III clinical trial [146, 147] |
Live attenuated vaccine | COVI-VAC | • Mild fever • Diarrhoea • Pain in arm |
• Show powerful immune and broader response. • Cellular immunity is long-lasting. • Highly qualified medical personnel are not required for administration. • Cost-effective. • Single-dose of immunization is sufficient to generate an immune response. |
• Requires cold storage, limiting the distribution. • Not appropriate for those with impaired immune response. • Substantial supplementary testing is required both in terms of safety and efficacy (Kaur & Gupta, 2020). |
Completed Phase I clinical trial [148] | |
Inactivated virus | 1. Coronavac 2. Covaxin 3. Sinopharm/BIBP 4. Sinopharm/WIBP 5. IMBCAMS 6. QazVac |
• Moderate fever. • Diarrhoea • Sleep quality decreases. • Pain in the arm • Inflammation at the site of injection. • Mild chest pain. • Mild fever. • Muscle pain • Redness in arm • Fatigue • Redness • Mild fever • Diarrhoea • Fatigue • Weakness • No such side effects |
• Simple platform and rapid development • The immune response is important • High induction of Nabs • Economical manufacturing • Stable at 2-8 degrees celsius. • Boost immune response. • Simple transportation and storage. • No harsh requirements. • It already has the necessary technology and infrastructure in place to support its development. • It can be used in conjunction with adjuvants to boost immunogenicity. • It can be used in people having compromised immunity. |
• High biosafety levels to be maintained. • Might result in hypersensitivity. • Huge quantities of viruses must be handled. • The integrity of the immunogenic particles has to be preserved. |
50.7% effective against symptomatic COVID-19 case in phase I/II 81% effective in Phase III clinical trial 79% effective against symptomatic cases 78.1% 73% 96% to 100% |
Entered Phase III clinical trial [149] Completed Phase III clinical trial Completed Phase III [150] Completed Phase III Completed Phase III Completed Phase III |
Subunit vaccine | 1. AdimrSC-2f 2. EpiVacCorona 3. UB-612 4. MVC-COV1901 5. NVX-CoV2373 (Novavax) |
• No side effects were reported. • No side effects. • No serious adverse effects • No observed side effects • Mild to moderate fever. • Weakness • Fatigue • Tiredness • Myalgia • Headache • Discomfort in hand. |
• The viral particle does not contain any living components. • Safe with very negligible side effects. • They regarded safe with people having impaired immune systems or with pregnant women. • Considered as safe. |
• Memory for future responses is questionable. • A repeated dosage is required. |
100% 89.3% |
Undergoing Phase 1 Completed Phase III clinical trial Undergoing Phase 2/3 Completed Phase II Completed Phase III [151, 152] |
10.2.1.1. Sixteen subunit vaccine candidates are in clinical trials and two are in phase II clinical trials:
• AdimrSC-2f was developed by Adimmune Biotech Corporation, Taiwan, and is currently in phase I of the clinical trial.
• EpiVacCorona was developed by Vektor State Research Center of Virology and Biotechnology, Russia.EpiVacCorona, consisting of the chemically synthesized antigen of SARS-CoV-2 protein associated with carrier protein and adsorbed on Aluminium Hydroxide.
• RBD-Dimer was developed by Anhui Zhifei Longcom Biopharma Institute of Microbiology 95. According to phases 1,2, and 3 clinical trials, ZF2001 induced higher neutralizing GMTs than those found in convalescent samples. The RBD-Dimer vaccine was approved in two countries for emergency use in the general population.
• UB-612 vaccine was developed by COVAXX, a US-based Company, and according to the phase 1 trials, UB-612 induces a high titer of neutralizing antibodies.
• MVC-COV1901 contains recombinant S- 2P antigen that was adjuvanted with CpG1018 and adsorbed with aluminum hydroxide. Medigen, Taiwan, developed the MVC-COV1901 subunit vaccine.
• NVX-CoV2373 was developed by Novavax Inc. NVX-CoV2373 shows 89.3% efficiency in its phase III clinical trial conducted in the United Kingdom and shows successful results in its phase 2b South Africa.
10.3. Nucleic Acid Vaccines
This platform is based on immunizing with nucleic acid-DNA or mRNA [112]. This platform offers significant flexibility in regards to antigen manipulation and high-speed development possibility. MRNA-based vaccine, mRNA-1273 by Moderna, completed phase 3 of clinical trials and showed vaccine efficacy of 94% [114]. Inovio Pharma International Vaccine Institute came up with a DNA vaccine candidate called INO-4800, which demonstrates a perfect safety profile and its vaccination generates both humoral and cellular responses, encouraging its continued development to prevent infection, disease, and death in the global population. Because of its high safety profile, it could become a favored vaccine choice for high-risk groups such as the elderly and those living with co-morbid conditions [115, 116]. BNT162b2 mRNA Vaccine by Pfizer-BioNTech completed its phase III clinical trial and was approved for emergency use in many countries. It is an mRNA vaccine encapsulated by lipid nanoparticles that encode the full-length spike protein of SARS-CoV-2. It is shown that two doses of BNT162b2 were safer over two months, delivering 95% efficiency against symptomatic SARS-CoV-2 infection in individuals 16 years or older [117].
10.4. Recombinant Vector Vaccines
This platform includes the usage of live viruses genetically modified to hold different pathogenic qualities, evoke an immune response, and code additional proteins [118]. This platform effectively generates immune response since it infects cells and survives in the body for a great deal of time, and can directly respond to the antigen-presenting cells [119]. The gene that encodes the spike protein may convey to the host cell via viral vectors. It exploits the viral vector’s ability to infect and transfer mRNA into the host cell.
10.4.1. Strategy for Vector Vaccine
The viral vector in which the gene is inserted may lose its ability to replicate. Primate’s virus from chimpanzees, humans, gorillas, etc., exploited as a viral vector.
There are various vector vaccines in advanced clinical phases. Out of these, four are currently in phase III clinical trial and approved for emergency use in the population.
10.4.1.1. Adenovirus from Chimpanzee:
ChAdOx1 is the vaccine candidate who used replication-defective adenovirus (recombinant) from chimpanzees, developed by the University of Oxford-Astra Zeneca, which completed the third phase of clinical trials and showed average vaccine efficacy of 70% [120].
10.4.1.2. Adenovirus from Gorilla:
GRAd-COV2 was developed by ReiThera, Italy. ReiThera vaccine was successfully entered phase 2/3 clinical phase. In the phase-I clinical trial, a single dose of GRAd-COV2 generated well neutralizing and spike binding antibodies and robust T cell response when administered all three doses.
10.4.1.3. Adenovirus from Human:
Ad5-nCoV vaccine candidate by CanSino Biologicals, China, is based on the adenovirus Ad5 (Human virus) constituted mRNA that encodes spike protein and shows the efficiency of 68.83% in preventing COVID-19 (symptomatic) two weeks after one dose and showed 65.28% after four weeks.
Sputnik V by Gamaleya Research Institute, Russia, is based on the two adenoviruses Ad5 and Ad26, which are administered sequentially, that contain mRNA which encodes spike protein. Sputnik V vaccine was 91.6% effective based on the number of SARS-CoV-2 infected patients from 21days after the first dose of sputnik V vaccine.
Ad26COVs1 by Johnson and Johnson, USA, is based on the human adenovirus Ad26. Ad26COVs1 was approved for emergency use and also authorized by FDA. The phase III clinical trial was conducted in eight countries and was shown to be 85% effective in preventing symptomatic SARS-CoV-2 infection.
10.4.2. Thrombosis Post-COVID-19 Vaccination
One of the recently recognized adverse events associated with adenovirus vector vaccines is TTS (Thrombosis-associated Thrombocytopenia Syndrome). The recombinant adenoviral vaccines such as ChAdox1 nCoV-19 (Oxford-Astrazeneca) and AD26.CoV2 (Johnson & Johnson) are being rolled to the masses to vaccinate against the ongoing pandemic. TTS, which was earlier known as VITT (Vaccine Induced Thrombotic Thrombocytopenia), causes venous and arterial thrombocytopenia along with rare cases of CVT (Central Venous sinus Thrombosis) and SVT (Splanchnic Vein Thrombosis). Both the vaccines use recombinant adenovirus vectors, which carry the genes encoding for the spike protein of SARS-CoV-2. Due to evident safety concerns associated with the vaccines, many European countries halted their administration to their citizens on 14th March 2021. Till the 4th of April 2021, 169 cases of CVT and 53 cases of SVT had been reported from ~34 million cases of ChAdOx1 nCoV-19 vaccine administrations in the EU [121]. US-FDA also halted the administration of AD26.COV2 after 15 cases of thrombosis were reported from 6.8 million doses [122]. In the UK, until 14th April 2021, 77 cases of TTS associated with CVT and 91 cases of thrombosis in other veins causing 32 deaths were reported from 21.2 million doses of ChAdOx1 nCoV-19. The benefits of the vaccine outweighed the risks of TTS, due to which Advisory Committee on Immunization Practices recommended resuming its administration for adults. The exact pathophysiology of TTS is not understood yet, but it involves the formation of antibodies that target platelet antigens. It is well known that adenovirus binds to platelets and causes activation. The activated platelets release reactive PF4 (platelet factor-4), which further attaches to the antibodies and induces the immune system to trigger even more platelets causing them to stick together. It forms blood clots and lowers the level of platelets. Such events are similar to those of HIT (Heparin Induced Thrombocytopenia), the critical difference being the non-requirement of heparin to activate platelets. Post-vaccination TTS development can be characterized if presented with arterial or venous thrombosis along with PFA-4 Heparin ELISA and mild-to-severe thrombocytopenia. Common post-vaccination symptoms, like fever, myalgias, headache, etc., which subside within 24-48 hours, are not indicative of TTS development. Instead, if these symptoms get severe and persistent for over three days with dyspnea, vomiting, chest or leg pain with swelling, it can be a possible case of TTS. Some recommended evaluation measures are a complete blood test, peripheral blood smear, D-Dimer, and PF-4 heparin ELISA. Current treatment measures use IVIG (IV-Immunoglobin) anticoagulation. It is advised to avoid the use of heparin and platelet transfusion [123].
10.5. Status of Vaccination for COVID-19 in India
The Indian government has constituted the task force, National Expert Groupon vaccine Administration for Coronavirus Disease, to provide guidelines on vaccine administration in India [124]. Based on the NEGVAC, the vaccines for COVID-19 will be administered first to frontline workers, healthcare workers, and individuals above 45 years, followed by individuals younger than 45 years that correlated with comorbidities. The government of India has set up a committee consisting of a panel of experts from various specialties, including nephrology, cardiology, oncology, and pulmonology, to decide the clinical criteria, based on which individual with comorbidities be prioritized first for vaccination.
The committee set up by the Indian government has recommended that individuals with kidney diseases, heart diseases that lead to hypertension, cancers, and sickle cell anemia should include in the priority list. The Indian government has decided to procure 600 million doses of the SARS-CoV-2 vaccines from vaccine manufacturers, like Pfizer, Moderna, etc. The government of India procured covishield, manufactured by Serum Institute of India, and covaxin manufactured by Bharat Biotech Ltd to administrated initially. Procuring of COVID-19 vaccine is the first requirement, but the distribution and vaccination to India's large population represent a considerable logistic challenge. The covishield manufactured by SII, a Pune-based company, was approved for limited (emergency) use by DGCI on January 2, 2021. Initially, the vaccine was approved in two doses 5-12 weeks apart, but after review by the expert committee, the period increases to 48-84 days.
On January 2, 2021, the DGCI's subject expert committee also approved Bharat Biotech's COVID-19 Vaccine “Covaxin” for emergency use in India. Covaxin has been recommending to be inoculated under clinical mode only. This vaccine was approved based on phase 3 immunogenicity data from 24K volunteers after the Ist dose of covaxin and 10K volunteers after the 2nd dose of covaxin. However, on March 3, 2021, the phase III clinical trial results of covaxin showed that it is 80.7% efficient in preventing SARS-CoV-2 infection. After review, the subject expert committee of DGCI gave authorization for emergency use and so that covaxin is no longer administered in clinical trial mode only.
Some vaccines are under development and, some are under production in other parts of the world that require storage conditions as low as -70oC but the vaccines produced by India for distribution require a storage temperature of 2-8oC only. The Indian government was constantly working on the logistics for the fast and effective distribution of the vaccines manufactured by Indian companies. The manufactures airlift the COVID-19 vaccines in the cold storage boxes with a digital temperature setup to four depots in Mumbai, Karnal, Kolkata, and Chennai. The government used the insulated van to transport COVID-19 vaccines to the designated places in the country.
10.6. Vaccine Durability and Future Challenges
The general concern with the vaccination process, also making it a significant challenge in corona viral infection, is that it might not induce long-term immunity so that reinfection might be possible. Vaccine success depends heavily on the vaccine target and platform. Hence choosing a suitable vaccine platform from all the available options, like nucleic acid, whole virus, subunit, or recombinant vector, is incredibly challenging [125, 126]. Finding undesired immune-potentiation that results in eosinophilic infiltration poses another challenge following test infection after immunization with vaccines [113]. The immune-escape variants have sparked questions about vaccine efficacy as the world ramps up SARS-CoV-2 immunization. COVID-19 vaccines have shown up to 95% effectiveness in reducing clinical events and up to 100% efficacy in preventing acute illness or hospital admission in environments with pre-existing variants. New variants that evade both normal and vaccine-induced immunity have raised questions about whether vaccines successfully prevent mild and severe COVID-19. A correlation of immunity against mild and severe SARS-CoV-2 infection would go a long way toward providing evidence for these decisions and overcoming the challenges that new variants are putting in the form of global SARS-CoV-2 regulation through widespread implementation of successful immunization [127].
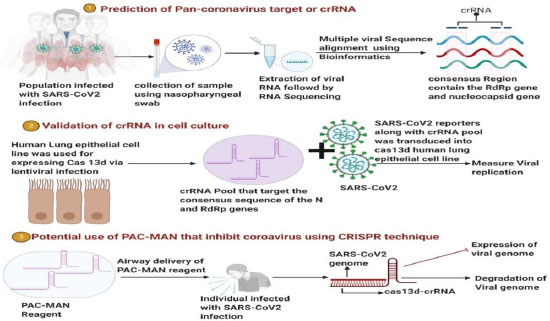
The short-term durability is likely to be adequate for slowing the spread of SARS-CoV-2 and resuming normalcy. However, the global spread of SARS-CoV-2 and the emergence of S protein variants and other variants across the globe can restrict vaccine efficacy. SARS-CoV-2 eradication from the population may be difficult due to concerns of unvaccinated and immunocompromised individuals. Since different mRNAs containing mutant S proteins can be rapidly synthesized and included within the LNP (lipid nanoparticle delivery system) carrier, the mRNA vaccine formulation is suitable for repeated or modified vaccination. In comparison, the AdV (adenovirus vector system) vector formulation induces AdV-specific immunity, which may restrict the effectiveness of repeated boosters due to immune-mediated vector clearance [128]. Vaccine development is an expensive process and usually takes years for it to be licensed [129]. Conducting integrated clinical trials, licensing safe and reliable vaccines in a transparent and accountable way, assessing effectiveness throughout (and after) vaccine deployment, providing equitable vaccine access globally, and so on are some of the additional problems that must be addressed [111].
11. CRISPR AS AN ANTIVIRAL STRATEGY TO COMBAT COVID-19
CRISPR is a tool used to edit, modify, or alter genomes. A group of scientists at Stanford University has aimed to utilize this technique to design broad-spectrum crRNA (CRISPR RNA), which should potentially inhibit all the types of coronaviruses [130]. A CRISPR-Cas13-based system, PACMAN (prophylactic antiviral CRISPR in human cells), was designed, as shown in Fig. (6). Class 2 types VI-D CRISPR-Cas13d system was employed to develop this therapeutic model, derived from Ruminococcus flavefaciens. The crRNA of Cas-13d has a customizable 22 nucleotide spacer sequence which directs the Cas-13d proteins to target RNA sequence with high specificity. This specific protein was chosen due to its small size, high specificity, and high catalytic activity in human cells. It has shown the potential to degrade viral RNA in SARS-CoV-2 and Human Influenza-A virus (IAV). In this highly conserved region, many sequences of the SARS-CoV-2 were screened. The virus strain used was synthesized. During their analysis, highly conserved regions between 47 SARS-CoV-2 strains, SARS-CoV and MERS-CoV, were found. Out of these, 47 were the two most highly conserved sequences coded for RdRp enzyme and nucleocapsid (N) protein. After a series of crRNA screening and matched, it was found that targeting of RdRp or N-protein was most effective by crRNA. Cas-13d functions by degrading or breaking down the target sequence present in the viral RNA. A guide RNA, that is synthesized, helps the protein in reaching its target site.
For the PACMAN system to work effectively, crRNA must be appropriately designed and present at a high concentration level. All known coronavirus sequences were analyzed to develop a pan-coronavirus inhibiting strategy, and all possible crRNAs targeting the genes were identified. A refined list of the minor groups of crRNA that could target all known coronavirus with zero mismatches was prepared. Six of the crRNAs showed the potential to target 91% of the coronavirus genome. While 22 crRNAs were seen to cover all known coronaviruses with zero mismatches. It included the beta coronaviruses, which cause SARS, MERS, and COVID-19. Hence, such information reflects a potential therapeutic area that has a broad-spectrum antiviral approach to most or all known coronaviruses. A significant drawback of the clinical use of PACMAN is the need for developing a safe and effective in vivo delivery system. This study using the Cas-13 variant to target viral RNA in human cells is one of its kind. So its efficacy against live SARS-CoV-2 strain is yet to be known [130]. A COVID-19 diagnostic tool kit using CRISPR technology has been developed by Sherlock Sciences, which has recently been given a EUA by the US-FDA [131].
12. NANODECOY BASED COVID-19 THERAPY
Nanodecoy is formed due to the fusion of cellular membrane nanovesicles recovered from human monocytes and ACE2 receptors expressed genetically engineered cells having an antigenic exterior of the SARS-CoV-2. When these are introduced into the mouse model, they compete with the host cells for binding to the virus, thereby protecting the cells from infection of the pseudo viruses and the SARS-CoV-2. Due to the expression of more cytokine receptors on the host cell's surface in severe infections, these nanodecoys infections, these nanodecoys effortlessly bind and neutralize inflammatory mediated cytokines, such as interleukin 6 (IL-6) granulocyte-macrophage colony-stimulating factor, thus avoiding IL 6 and GM-CSF to attach to the host receptors and significantly suppress immune disorder and lung injury in acute pneumonia [132].
13. GOLD NANOPARTICLES FOR PREVENTION OF SARS-CoV-2 ENTRY TO HOST CELL
Along with virus aggregation, gold nanoparticles are not only responsible for SARS-CoV-2 accumulation that leads to the death of the virus, but they can directly disrupt the cell entry process [133]. SARS-CoV-2 contains a heptad repeat 1 (HR1), a heptad repeats 2 (HR2), and a fusion protein (FP). Following the FP insertion into the cell membrane, HR1 and HR2 bind to form a six-helix bundle (6-HB). The 6-HB promotes cell fusion after binding SARS-CoV-2 envelope and host cell membrane. However, a peptide named pregnancy-induced hypertension (PIH) mimics the HR2 conformation and binds with the HR1 to block the production of 6-HB, thereby inhibiting the cell fusion process. This is a standard natural method of stopping the virus entry. When gold nanorods (PIH–AuNRs) interact with this peptide, bind, and get immobilized on the surface, there was a 10-fold higher inhibitory activity which might obstruct SARS-CoV-2 cell fusion to host cell and that too with an optimized condition not affecting the host cell [133].
14. SILICON AND SELENIUM NANOPARTICLES ALSO PREVENT VIRUS ENTRY TO A HOST CELL
Porous silicon NPs (SiNPs) are particularly appealing due to their high biocompatibility and biodegradable nature. These NPs gradually liquefied into the water and converted into nontoxic silicic acid [134]. Mesoporous-SiO2 (mSiO2) NPs, when combined with various antiviral drugs, exhibit the ability to stick themselves to the enveloped viruses via hydrophobic or hydrophilic interactions. The strong bond formed between the functionalized mSiO2 NPs and the virus hampers the attachment of the virus to the host cell receptors and reduces the viral entry into the cells [135]. Selenium being biocompatible like silicon in nanoparticles is present in several selenoproteins. It is crucial for biological processes and presents various antiviral effects at high concentrations. These NPs are combined with multiple antiviral drugs, like Arbidol (ARB), which effectively block entry of the influenza virus and reduce viral infection, thereby reducing host cell apoptosis [136].
CONCLUSION
The SARS-CoV-2 infection spread rapidly among the world population after it emerged from Wuhan, China, in December 2019. WHO declared COVID-19 as a worldwide pandemic, and there is still no proven effective drug available to treat COVID-19 disease. Even though several vaccines have been developed, the long-term effects of these vaccines are unknown. In this article, we highlight the potential therapeutics that can be beneficial in treating the disease caused by SARS-CoV-2 based on their prior, effective use against other RNA viruses and current clinical trials. It may be tremendously advantageous for our healthcare system to repurpose licensed medications and experimental compounds that can block the replication and assembly of the SARS-CoV-2. Several repurposed drugs are underway in the clinical trials to determine their potential efficacy. The 2019-nCoV results in elevated amounts of cytokines and chemokines, and this atypical release of pro-inflammatory factors cause hyper-inflammation. Subsequently, this may lead to apoptosis of lung epithelial and endothelial cells, thus causing vascular leakage, hypoxia, and edema, which are still unsolved problems in these patients. Therefore, it is necessary to consider inflammation management and immune modulation as essential steps. Even though various vaccines have been launched for public use, and many are under trials or preclinical trials, it is too early to say whether they will prove effective and safe.
Moreover, vaccine development in this pandemic has been an accelerated process that bypassed various stages of testing; however, vaccine licensing is a long shot. Since no approved drugs are presently available for the treatment, it becomes necessary to use safe and effective options that reduce the severity of the infection, thereby increasing the chances of survival.
AUTHORS' CONTRIBUTIONS
AY contributed to data curation, conceptualization, figure illustration, investigation, writing, reviewing, and editing. SS, NB, and SG participated in writing, reviewing, and editing. VS, PS, and HK contributed to conceptualization, visualization, investigation, writing, reviewing, and editing.
CONSENT FOR PUBLICATION
Not applicable.
FUNDING
None.
CONFLICT OF INTEREST
The authors declare no conflict of interest, financial or otherwise.
ACKNOWLEDGEMENTS
The authors would like to acknowledge Sr. Copyrights Coordinator-Copyrights Team, ELSEVIER, for permitting to reproduce figure 6 and thank the copyright clearance center of the journals for not requiring the permission to reproduce article materials. All these Figs were reproduced using BioRender. The authors have included citations in reference.