All published articles of this journal are available on ScienceDirect.
Nanotechnology-Based Weapons: A Potential Approach for COVID-19
Abstract
In the last few decades, viral infections have caused a greater number of health constraints worldwide. This havoc has created challenges for the healthcare system. Since the pandemic began, COVID-19 has killed more than 2.5 million people across the world. We are still witnessing deaths daily due to the deadly virus SARS-CoV-2 which is the causative agent for COVID-19. Thus, there is an increasing concern about finding an apt way to control the spread of this virus. Recently, the application of nanotechnology-based approaches has emerged as a ground-breaking step in the medical sector owing to their potential for accurate diagnosis and specific treatment in a wide range of health problems, including viral diseases. Therefore, the implementation of nanotechnology can be an articulate strategy to confront the rising distress of COVID-19. The present review particularly emphasizes the perception of several nanoformulation-based approaches as an appropriate means to safeguard mankind against COVID-19.
1. INTRODUCTION
Recently, novel coronavirus or severe acute respiratory syndrome coronavirus 2 (SARS-CoV-2), the causative factor of the COVID-19 disease, has jolted the entire world with its severity causing widespread morbidity and mortality [1]. The disease started in Wuhan city, China, in December 2019 and imposed a major public health crisis. Primarily it was classified as an outbreak of pneumonia of unknown aetiology that was subsequently declared by the World Health Organization (WHO) as a pandemic in March 2020 [2]. As of now, the current priority of the research fraternity is to look into novel therapies of high effectivity and smart diagnostics tools for an early-stage prognosis and monitoring of COVID-19. After much permutation and combination, researchers have identified chloroquine, antivirals (e.g. Remdesivir and Interferon), and convalescent plasma treatments as an effective means to tackle the exotic virus [3]. These drugs have proved to be an effective mode of treatment in many critical cases, but they cannot be considered the gold standard against the SARS-CoV-2 owing to many drawbacks. An ideal drug for targeting the SARS-CoV-2 should selectively inhibit virus progression by affecting one or more proteins in the life cycle or kill the SARS-CoV-2 virus selectively. To suffice the above criteria many therapeutic agents are scrutinized such as antibodies, silencing RNA (siRNA), antivirals, repurposed drugs, phytochemicals, etc. However, each of these has some limitations which flounder their effectiveness. Similarly, conventional diagnostic methods for COVID-19 such as Reverse Transcription-Polymerase Chain Reaction (RT-PCR) and Computed Tomography (CT) scan are also plagued by shortcomings of time consumption, high cost, and requirement of extensive laboratory facilities [4]. The different diagnostic measures taken for the detection of COVID-19 are mentioned in Fig. (1). Hoffman et al. have assessed a commercially existing test established for rapid diagnosis (~15 minutes) of SARS-CoV-2-specific IgM and IgG by 29 PCR-confirmed COVID-19 cases and 124 negative controls. In one of their results, where they have showed the IgM and IgG reactivities in PCR-confirmed COVID-19 patients. The result showed the number of PCR-positive cases positive or negative for IgM or IgG based on the number of days after onset of COVID-19 symptoms (Fig. 2) [5]. To identify the number of affected cases, some of the U.S Food and Drug Administration (FDA) - Emergency Use Authori- zation (EUA) approved COVID-19 detection tool kits are used (Table 1). Such a time-consuming scenario demands the development of speedy, pocket-friendly, and prompts diagnostic methods.
Nanotechnology is the field of science that has revolutionized the health sector landscape which is rapidly expanding and making its presence felt in many aspects of modern life [6]. Nanotechnology-based approaches are very powerful in vaccine development and immunoengineering, as nanoparticles and viruses act at the same scale. Therefore, nanotechnology can be aptly implemented against viruses as the latter is nothing but “smart and able nanoparticles”. Numerous nanotechnology-based tools have shown promising efficacy to combat several viral pathogens such as human immunodeficiency virus (HIV), human papillomavirus (HPV), Herpes Simplex Virus (HSV), and respiratory viruses in preclinical investigations [7]. Nanomaterial-based delivery formulations favour the presence of an optimum concentration of these drugs in the body, preventing systemic toxicity and untimely degradation [8]. Similarly, another strategy used for COVID-19 therapy is to control the cytokine storm in patients. In this context systemic delivery of nanoparticles made of Polyethyleneimine (PEI) or poly (lactide-co-glycolide) (PLGA) or Polyethylene Glycol (PEG) - poly (ε-caprolactone) (PCL) loaded with interleukins could be highly beneficial for controlling the cytokine storm. The brightest propositions of nanotechnology for fighting COVID-19 are the vaccine candidates, where nanoparticles are used as carriers and/or adjuvants. Previously, nanoparticle-based formulations have been implemented for vaccination through intranasal routes against respiratory viruses such as influenza and coronaviruses [6]. In this regard, most of the vaccines in the advanced stage for prevention of COVID-19 are nanobased vaccines. The present arsenal of COVID-19 management strategies is further strengthened by incorporating nanoparticle technology in respiratory masks for enhancing high breathability and filtration efficacy or for fabricating Personal Protective Equipment (PPE) [8]. Furthermore, nanoproducts can also act as efficient disinfectants or as self-surface sanitizers like Cu2+ and Ag+ nanoformulation or as nanosilver-incorporated soaps, etc. [9-11]. As mentioned above, besides therapeutics, the key part for the management of the COVID-19 pandemic lies in designing smart diagnostic systems that are not only required for disease prognosis but also for understanding the virus pathogenesis and progression. In this context, biosensors are being explored as an impactful diagnostic tool owing to their sensitivity and accuracy for the detection of SARS-CoV-2. These diagnostic agents have already been implemented for the successful detection of viruses like Surface Acoustic Wave (SAW) sensors for Ebola antigens [12] and aptasensors for avian influenza virus, H5N1 [13]. Further, genosensors are also being implicated to differentiate genetic material of Zika virus from dengue (DENV) and chikungunya (CHIKV) viruses in serum, urine, and saliva samples [14]. In this light, G-quadruplex-based electrochemical and optical biosensors have proved to be effective in the detection of a wide spectrum of pathogens including SARS-CoV-2 [15]. To quickly recognize the infection or immunological response, sensitive nanobased sensors are also designed for high accuracy. Recently, several nanoenabled biosensors based on Field-Effect-Transistor (FET) or dual responsive photo-plasmonic using Internet of Things (IoT) technology or Artificial Intelligence (AI) have been fabricated for COVID-19 diagnostics by overcoming the bottlenecks of conventional diagnostic agents [16].
The present review provides a comprehensive understanding of the COVID-19 pandemic, as well as sheds light on the possible utility of these nanosized materials for the diagnosis and treatment of COVID-19. Thus, this may open new avenues for nanotechnology-based application as weapons against COVID-19 disease.
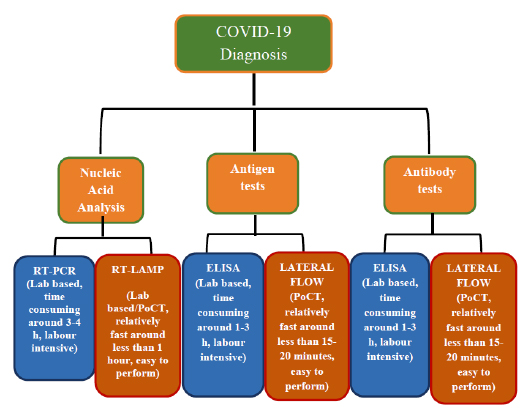
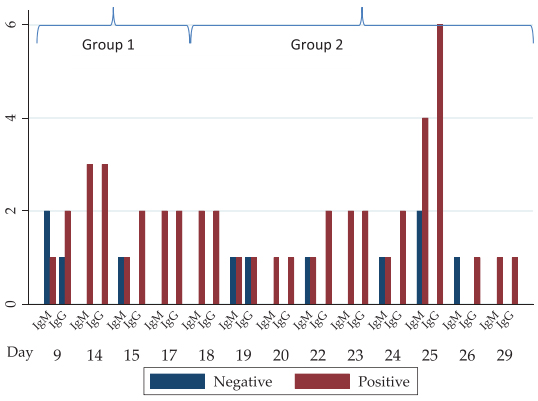
2. ABOUT SARS-CoV-2 AND COVID-19 DISEASE
SARS-CoV-2 shows maximum resemblance with the known Beta coronavirus in bats [17]. However, the role of SARS-CoV-2 in contributing to any previously known human cases is not clear [18, 19]. Ample studies are ongoing to know the vulnerability of SARS-CoV-2 in different species of animals [20, 21]. Moreover, a recent study proposes that SARS-CoV-2 infected humans can transmit the infection to other mammals, such as dogs [22], cats [23], and farmed mink [24]. Further, the risk of transmission of infection from these infected mammals to humans is still unknown. Few characteristics features of the virus, mechanism of transmission, and biological interference of the virus are discussed below.
2.1. Salient Features of the Virus Family
Coronaviruses are one of the types of infectious pathogens that have been found in the world a decade ago. The first coronavirus was isolated from chickens in 1937 [25]. In 1960 the first human coronavirus was identified [26]. Currently, the COVID-19 pandemic is caused by the SARS-CoV-2 virus, a new species that infects particularly humans. The virus belongs to the family of Coronaviridae [27]. This virus family is characterized by enveloped, single-stranded and positive-sense RNA. The genome size of the virus is 30 Kb, which consists of a 5'-terminal noncoding region, an open reading box (ORF) 1 a/b-coding region, the spike glycoprotein (S protein) encoded by s region, an envelope protein (E protein) encoded by an e region, membrane protein (M protein) encoded by m region, a nucleocapsid protein (N protein), encoded by n region and a -3'-terminal noncoding region [28]. During virus attack, the spike glycoprotein S, precisely binds to the surface receptor of the vulnerable host cell. The virus envelope is formed by the M and E proteins, whereas in the assembly of the virus, N protein is mainly involved. The coronavirus family is divided into four genera such as α, β, γ, and δ based on the structure of the genome and phylogenetic analysis of the virus [29] (Fig. 3). Out of these four genera, the first two α and β infect mammals and humans whereas, the other two infect the birds. SARS-CoV-2 belongs to the β genus. The shape of this virus is round or oval having a diameter of around 60-140 nm which gives the appearance of crown shape under an electron microscope [30]. Other six different types of coronaviruses like coronavirus 229 (HCoV-229E), Human coronavirus OC43 (HCoV-OC43), Human coronavirus NL63 (HCoV-NL63), Human coronavirus HKU1 (HCoV-HKU1), SARS-CoV, and the Middle East Respiratory Syndrome coronavirus (MERS-CoV) also infect humans [31]. The amino acid sequence analysis of a protein depicts around 94.6% similarity among these seven conserved non-structural proteins between SARS-CoV-2 and SARS-CoV. Moreover, the genome of SARS-CoV-2 and bat SARS-like coronavirus shows around 96% similarity [32]. These coronaviruses are heat and ultraviolet rays sensitive. These viruses can be stored at -80 °C for a longer time and can also be inactivated for 30 min at 56 °C. Further, SARS-CoV-2 can be effectively inactivated by using 75% ethanol, peracetic acid and chlorine-containing disinfectants [33].
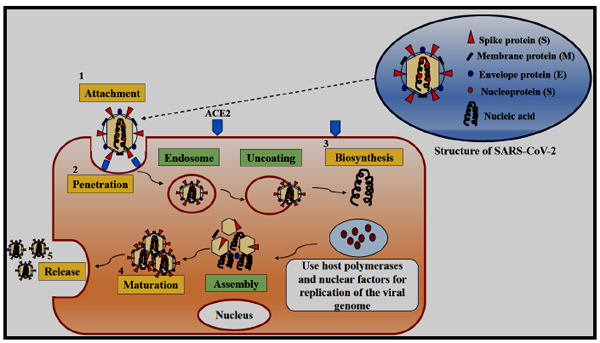
2.2. Biological Interaction of SARS-CoV-2 in Host Cells
Coronaviruses can infect a wide variety of host species. The virus life cycle inside a host generally comprises 5 steps such as attachment, penetration, biosynthesis, maturation, and release [34]. First, the virus binds to the host receptors which are known as attachment, and then it enters the host cells by the process of endocytosis or membrane fusion called penetration. After penetration, it releases its genomic content into the host cells, replicates within the host cells, and synthesizes its viral proteins through the biosynthesis process. Thereafter, the new viral particles are made in the maturation phase which are finally released. A transmembrane trimetric glycoprotein known as spike protein bulges from the virus surface which determines the coronavirus types and their selection towards the host. Spike proteins have two functional subunits; the S1 subunit, which is responsible for host cell receptor binding whereas the S2 subunit is required for the virus fusion with the cell membranes. Angiotensin-Converting Enzyme 2 (ACE2) is reported to be the receptor for SARS-CoV-2 [35]. The ACE2 expression is high in the heart, ileum, kidney, and bladder, further also very high in lung epithelial cells. When the virus binds to the host protein it leads to protease-mediated cleavage of the spike protein in two sequential steps for activating the same. The cleavage model states that cleavage occurs at S1/S2 site for priming and activation at the S′2 site housed in the adjacent position to a fusion peptide within the S2 subunit. The subunits like S1 and S2 remain non-covalently bound at the cleavage S1/S2 site. The S1 subunit contributes to the stabilization of the S2 subunit at the perfusion state. Subsequently, the S2 cleavage site leads to the activation of spike protein that helps in conformational changes for irreversible membrane fusion [36]. The coronavirus spike is uncommon amongst viruses, as varieties of different proteases are involved in cleaving and activation. The characteristic exclusive to SARS-CoV-2 amongst coronaviruses is the presence of a furin cleavage site (“RPPA” sequence) at the S1/S2 site. The cleavage pattern of SARS-CoV spike protein is different from that of SARS-CoV-2 as in the former case the assembly occurs without the process of cleavage [36]. The cleavage process occurs at S1/S2 site by using other proteases such as cathepsin L and transmembrane protease serine 2 (TMPRSS2), but the virus remains very pathogenic due to the ubiquitous expression of furin. Men are reported to be expressing a high amount of ACE2 receptors in their alveolar cells as compared to women. Moreover, Asians are normally expressing higher ACE2 receptors in their alveolar cells than the White and African American people [37]. After binding of SARS-CoV-2 with the ACE2 receptors, the level of ACE2 expression increases which leads to alveolar cell damage. The alveolar damage in turn leads to activation of a series of systemic reactions and eventually death. The higher susceptibility of Asian males towards the coronavirus infection is also confirmed. Wrapp et al. have reported a 10 to 20 times stronger binding efficacy of SARS-CoV-2 to ACE2 receptor than that of the SARS-CoV [38].
2.3. Symptoms of SARS-CoV-2 and its Mechanism of Transmission
This virus mainly causes infection in the respiratory tract and intestine of humans and animals respectively. As a result, patients show symptoms like fever, dry cough, runny nose, sore throat, and diarrhoea. A few patients are also observed with dyspnoea, while others can develop acute respiratory distress syndrome, coagulation dysfunction, and septic shock [39]. From the presently available cases, it was observed that the mild patients showed low fever and slight fatigue but with no pneumonia whereas, other patients with chronic diseases show poor prognosis and develop pneumonia [40]. Pathological investigations of COVID-19 indicate a decrease in CD4+ T cells, CD8+ T cells, B cells, and natural killer (NK) cells along with an increase of pro-inflammatory cytokines like interleukin-6 (IL-6), tumor necrosis factor (TNF) and IL-1, and chemokines (IL-8). Enhanced level of pro-inflammatory cytokines causes state known as the cytokine storm syndrome which is also responsible for the severity of COVID-19 infection [41].
It has been accepted that SARS-CoV-2 is mainly transmitted by the inhalation of infectious aerosols and its incubation period is around 2-14 days [42]. Mainly the SARS-CoV-2 transmits through respiratory droplets of infected people as well as from things contaminated with virus droplets. Moreover, some studies have illustrated that the infective viral particles present in faeces and urine can also be responsible for the transmission of the disease [43]. A clinical report from China revealed that there was a detectable level of RNA in the faeces of 3 children during recovery of COVID-19, despite their negative nucleic acid reports from throat swabs [44]. In another clinical study, Chen et al. reported that 18 patients showed viral shedding in faeces for a week even after negative conversion in pharyngeal swabs [45]. A recent meta-analysis study illustrated the occurrence as well as shedding of faecal RNA in 27% of patients with COVID-19 [46]. Hence, transmission is not only by the nucleic acid of the virus, but also it could be faecal-oral; because its detection in faeces suggests that there are infective viral particles. However, there is not enough evidence available yet and more studies are required to confirm the faecal-oral transmission of the virus.
3. ROLE OF NANOTECHNOLOGY IN VIROLOGY
Several viral diseases have emerged in the last few decades which have now established their roots in human populations worldwide. Antiviral drug therapy is the best way to deal with this problem. However, these drugs are still limited concerning number and variety. Besides, conventional antiviral therapy deals with a narrow therapeutic range, a requirement of high doses, frequent administration due to their limited aqueous solubility, short half-life, and/or slow uptake by the body tissues [47]. Prolonged antiviral therapy is required due to the physicochemical limitations of the drug which results in the development of drug-resistant virus strains and enforces daily repeated administration. As the development of new antiviral drugs is quite challenging, researchers are exploring new perspectives for the treatment of viral diseases by enhancing the efficacy of existing antiviral drugs. With the advent of nanotechnology, interest in nanoparticles as drug delivery vehicles have grown leaps and bounds owing to their versatility and wide application range. Nanotechnology is the creation and utilization of the material and system on the nanometre scale (a nanometre is one-billionth of a meter). The medicines which are formulated in the nanometre range are called nanomedicine [48-50]. Nanoformulations show the potentiality for better drug delivery with a simultaneous aim of fewer side effects towards the patient. Improving treatment success for viral diseases has become a fundamental responsibility. In this context, nanotechnology-based formulations possess unique characteristics such as (i) small size, (ii) large surface area to volume ratios (for incorporation of high drug load), (iii) tunable surface charge for facilitating cellular entry across the negative cellular membrane, (iv) biomimetic properties which result in inherent antiviral properties, (v) anchoring ability for targeting moieties to increase specificity for cell types, tissues and cellular components (vi) enhanced solubility with better pharmacokinetic and/or pharmacodynamics property permitting a higher accumulation with controlled and sustained release, (vii) enhanced protection of the entrapped drug from the harsh physiological environment (viii) reduced toxicity, (ix) flexibility for multifunctionality to stimulate replication of the hidden virus and deliver an antiviral to the activated cell [51]. Numerous diverse nanoplatforms such as polymeric, liposome, micelle, and metal-based nanoparticles of different compositions can be used for the entrapment of appropriate antiviral drugs for viral therapy (Fig. 4). Potential boosted progress made by nanotechnology-based antiviral therapy creates great expectations for innovative new therapeutic strategies towards attacking or eradicating viral disorders. Nanotechnology was recently recommended to deal with SARS-CoV-2 infection. The use of nanotechnology is not limited to their use as antiviral or as drug delivery vehicles for these antivirals. Some of the different ways in which the nanotechnological approach can ensure better management of SARS-CoV-2 infection are discussed discreetly here.
4. POSSIBLE NANOTECHNOLOGY-BASED WEAPONS FOR TACKLING COVID-19
Recent global epidemics of coronavirus have emerged unexpectedly and have spread like wildfire. Management of any disease depends upon its severity, its mode of transmission, appropriate steps taken to minimize the transmission of infectious diseases [52]. The nanotechnological tools can be implemented in the three pillars for disease management (A) For diagnosis (B) As treatment modalities (C) As control and prevention methods (Fig. 5).
4.1. As Diagnostic Agents
An accurate, rapid and early diagnosis of COVID-19 is vital as it can alter the course of therapeutic efficacy and effectiveness of the treatment. WHO and other organizations have confirmed that a rapid diagnostic tool is indeed very vital to end this pandemic and in this context, nanoparticles can be very handy in developing such diagnostics agents, some of which are mentioned below.
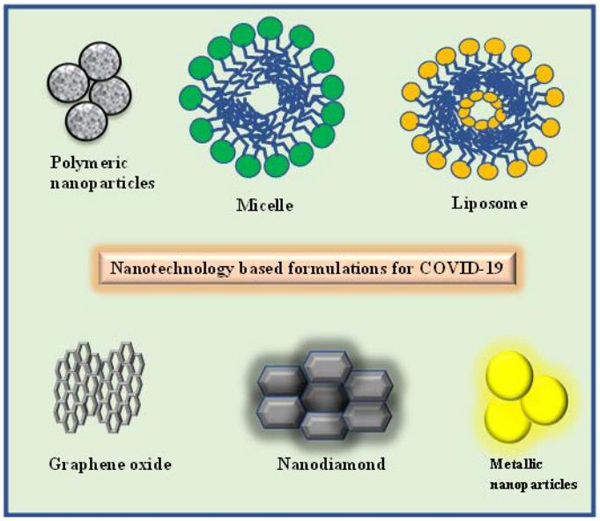
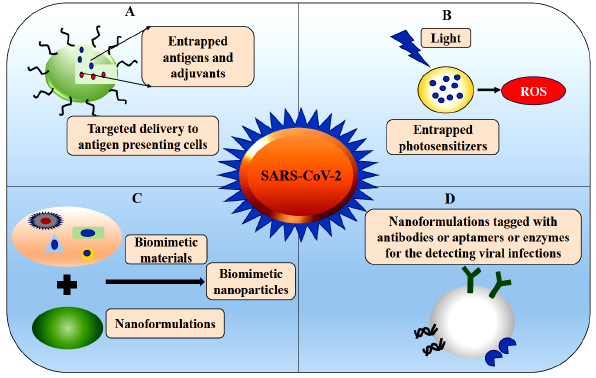
4.1.1. Nanobiosensors as Tools for Clinical and Environmental Analyses of SARS-CoV-2
Nanobiosensors have combined features of optical and electrical properties of nanomaterials and receptors of biological or synthetic molecules that are used for selective detection of any kind of analyte. Through nanobiosensors, detection of biological molecules such as antibodies micelles, metabolites, nucleic acids, and pathogens, etc. can be done. This type of detection technique can be applied to detect viruses with known antigens and available antibodies. In this context, many researchers have come up with unique schemes for detecting the spike protein which is a characteristic feature of the SARS-CoV-2 virus. Recently, detection of SARS-CoV-2 in culture medium is done through FET (graphene-modified sheets) by using a precise antibody for SARS-CoV-2 spike protein in culture medium as well as in clinical samples [53]. As biosensors were found to be effective in detecting respiratory viruses, in an independent study, Peng et al. formulated a phase-modulation plasmonic biosensor working in the near-infrared (NIR) regime, which can also be useful for the detection of SARS-CoV-2 and its spike glycoprotein [54]. Similarly, FET-graphene-coated biosensors were developed for identifying SARS-CoV-2 spike protein [53]. A plasmonic biosensor that utilizes gold nanorods (Au NRs) for the detection of COVID-19 SARS-CoV-2 spike protein was demonstrated by Das et al. [55].
In the context of nanobiosensors, paper/nanomaterial-based sensors are currently the most described testing mode for analyzing COVID-19 related biomarkers that can be observed with the naked eye or can be seen through a smartphone [56, 57]. Similarly, emerging plug-and-play diagnostics to manage the SARS-CoV-2 will be highly useful in future epidemics. Jang et al utilized a GO-based assay for screening selective inhibitors of a hepatitis C virus (HCV) helicase along with inhibitors of SARS-CoV helicase. This particular screen was able to find five inhibitors that are extremely selective for the HCV helicase orthologous to the SARS-CoV helicase. Some of these hits were assayed through GO-based assay as the single-stranded nucleic acids can bind to the GO surface via π−π stacking interactions among the hexagonal graphitic units of GO and the nucleotides [58].
Transition Metal Dichalcogenides (TMDCs) are comprised of hexagonal layers of metal atoms (M) that are sandwiched between two layers of chalcogen atoms (X) with an MX2 stoichiometry. It has morphological variability which is currently fascinating because of its greater surface area and edge reactivity. To anchor biologically relevant molecules, S and Se termination of the basal plane and edges are particularly favourable. Nanoflowers of TMDCs are presently attracting therapeutic applications since they can host a huge number of molecules and nanoparticles on their edges to detect, deliver molecules, inactivate pathogens, and interfere with cellular functioning [59]. The TMDC based nanomaterials have better stability in the air; with a suitable substrate anchoring it makes them highly useful as aerosol on surfaces or face masks for the detection and inactivation of the virus [60].
4.1.2. Metal Nanoparticles as Diagnostic Agents
Metal nanoparticles possess brilliant optical properties, inherent photostability, high extinction coefficient, and localized surface plasmon resonance effect. Further, metallic nanoparticles due to their unique physicochemical characteristics could address all the critical requirements for developing clinically translational diagnostic agents used for the identification of SARS-CoV-2. Recently, gold nanoparticles (AuNPs) have been used as a diagnostic agent where a colorimetric assay based on these particles was designed using thiol-modified antisense oligonucleotides particularly against nucleocapsid phosphoprotein of SARS-CoV-2. These modified nanoparticles helped in the rapid detection of positive COVID-19 cases within 10 minutes from the isolated RNA samples [61]. Similarly, a lateral flow assay was developed using lanthanide-doped polystyrene nanoparticles (LNPs) by Chen et al. for the detection of anti-SARS-CoV-2 IgG in human serum [62]. Another metallic nanoparticle that has been handy as diagnostic tools is the magnetic nanoparticle. This nanoparticle was coated with poly (amino ester) and carboxyl groups for the development of a sensitive detection method based on RNA extraction of SARS-CoV-2 [63].
4.2. As Treatment Modalities
There are several ways in which nanomaterials are used as facilitators of treatment modalities against COVID-19.
4.2.1. Nanoparticles as Antiviral Agents
Nanotechnology-based antiviral formulations can be inorganic, organic, metallic, and hybrid. Silver nanoparticles (AgNPs) are the most intensely investigated nanotechnology-based strategy for the treatment or detection of viral diseases such as Human Immunodeficiency Virus (HIV), Herpes Simplex Virus (HSV), Feline Calicivirus, Poliovirus, Influenza virus, etc. Lara et al. demonstrated the potential application of AgNPs as a strong antiviral agent against viral infection. AgNPs interact with the viral gp120 thereby inhibiting the pathogenesis in both cell-free and cell-associated viruses [64]. In another study, Vijayakumar and Ganesan et al. showed the efficacy of polyethylene glycol encapsulated AuNPs against HIV-1, which might block the attachment of gp120 with CD4, resulting in viral entry inhibition [65]. The use of magnetic nanoparticles as antivirals against bacteriophages, such as MS2 and other highly infectious viruses like Zika virus, HCV, H5N2, has been reported [66]. Amine functionalized polymeric gelatine nanoparticles loaded with zidovudine efficacy were evaluated against HIV which illustrated promising biocompatibility and antiviral activity [67]. Gao et al. developed a nanostructured lipid formulation containing podophyllotoxin (POD) which reported efficient drug release and hemocompatibility and demonstrated viral inhibition in VK2/E6E7 cell lines challenged with HPV [68]. Dendrimers conjugated with therapeutic agents showed good antiviral activity against some viruses such as influenza virus, HSV-1, 2, HIV, Ebola virus, Zika virus [69, 70]. In another study, acyclovir-loaded nano-niosome against HSV-1 showed promising antiviral activity with improved delivery in vitro [71, 72].
The recent success of nanoparticles on respiratory viruses, including SARS-CoV has prompted its use against SARS-CoV-2. Very recently, Sundararaj et al. explored the role of AgNPs against SARS-CoV-2. He and his group studied the efficacy of AgNPs of different sizes and concentrations and stated that nanoparticles of diameter around 10 nm at a concentration of 1 and 10 ppm were effective in inhibiting extracellular SARS-CoV-2 while the particles were toxic at a concentration of 20 ppm [73]. In another study Zhang et al., successfully formulated nanosponges from the plasma membrane of the human macrophages and type II alveolar epithelial cells and used these engineered nanoparticles to neutralize SARS-CoV-2 in vitro conditions [74]. Similarly, a molecular docking study using Dissipative Particle Dynamics (DPD) simulations was used to theoretically design Angiotensin-Converting Enzyme Inhibitor (ACEI) decorated remdesivir-loaded PLGA nanoparticles (NPs) against SARS-CoV-2 [75]. All these initial studies hint at the success of nanoparticles for tackling the COVID-19 pandemic which can further be explored to find the ideal solution to the present problem.
4.2.2. Role of Nanoparticles Controlling the Cytokine Storm
SARS-CoV-2 when housed inside the human lungs spreads its infection rapidly, and triggers a cytokine storm also known as Cytokine Release Syndrome (CRS), which results in severe deterioration of a patient's health due to excessive immune response. This inflammatory storm is the vital cause of Acute Respiratory Distress Syndrome (ARDS), which is chiefly connected with the failure of multiple organs and remains as a significant cause of death in critical patients [76]. An elevated IL-6 level was observed in patients with SARS and was correlated with disease severity. Although the receptor of IL-6 (Tocilizumab, an anti-IL-6 receptor antibody, and sarilumab) or IL-6 itself (siltuximab) can be used for therapy as immune checkpoints [77, 78] they face some challenges. Therefore, designing therapeutic strategies which can suppress the cytokine storm is highly essential. Nanomaterials' usefulness has been used to regulate the immune response to an optimized level and to inhibit cytokine release [79]. The probable nanosystems can specifically improve the effectiveness of the delivery of immunosuppressants into the target immune cells, with reduced drug dose and possible side effects. Chen et al. in their study found the presence of ACE2-expressing CD68+, CD169+ macrophages containing SARS-CoV-2 nucleoprotein antigen which exhibited an augmented release of IL-6 [80]. With the aid of a nanoformulation-based approach, it can be targeted towards specific immune subpopulations thus avoiding complications. Similarly, various research groups have prepared and explored different nanomaterials for their impact on different immune cell subpopulations [81, 82]. Pentecost, et al. in a study reported that a low dose of dexamethasone-adsorbed nanodiamond functionalized with octadecylamine helps in anti-inflammatory and pro-regenerative activities in human macrophages in vitro, whereas in mice there was reduced macrophage infiltration and expression of proinflammatory mediators like nitric oxide synthase (iNOS) and tumor necrosis factor-alpha (TNF-α) [83]. The formulated nanodiamond particles propose their usefulness as an inherently immunomodulatory platform.
Upon administration, nanoparticle interactions take place with numerous components of the immune system either to augment or impede its role [84]. Cytokines are proteins formed by different kinds of cells including immune cells in response to activation. These cytokines play a major role in maintaining homeostasis both by controlling and regulating the immune response in the form of cytokine network interactions. Survivability of the patients can increase significantly in early-stage sepsis when pro-inflammatory cytokines are quickly and completely removed from the bloodstream. Most often the proinflammatory cytokines are measured to foresee the immunomodulatory effects of nanomaterials [85]. Sandeman et al. used hierarchical porous carbide-derived carbons with tuned porosity for the removal of many cytokines including IL6, TNF-α from blood plasma [86]. Seredych et al. used poly(tetrafluoroethylene) granulated graphene nanoplatelets having high adsorption capacity for rapid proinflammatory cytokine removal that prevents death arising from an uncontrolled inflammatory cascade, allowing enough time to fight the virus by the defence mechanisms of the human body [87]. Such a strategy can be very well exploited to control the cytokine storm in patients affected with COVID-19.
4.2.3. Photodynamic and Photocatalytic Inactivation of SARS-CoV-2 Using Nanoparticles
Photodynamic therapy (PDT) is an exceptional tactic to inactivate SARS-CoV-2. It is a light-based method that can attack the target cells by exciting the photosensitive agents (called photosensitizers) and generating reactive oxygen species leading to cell death. PDT was first used clinically to eradicate viruses by local treatment [88]. Further, PDT-based virotherapy against diverse viruses such as HPV, HSV, and HIV is reported earlier [89, 90]. Although PDT is quite an efficient approach, its clinical use is restricted due to hydrophobicity of photosensitizers, reduced target specificity, and inadequate tissue penetration capacity. In an aqueous solution, the aggregation property affects its photochemical and photobiological properties. To tackle this problem, Lim et al, developed and proposed photodynamic inactivation of viruses with Up Conversion Nanoparticles (UCNPs) using sodium yttrium fluoride (NaYF4) with zinc phthalocyanine grafted onto it. These nanoparticles are further coated with polyethylenimine (PEI) for hydrophilicity. These UCNPs are highly effective against the dengue virus and adenovirus type 5 [91]. Apart from this, Fullerene and graphene are also ideal candidates against different viruses [92]. Similarly, graphitic carbon nitride, MXenes, black phosphorus, tungsten disulfide, and molybdenum disulfide, which have shown better performance for improved efficacy in cancer therapy, can be beneficial determinants to inactivate SARS-CoV-2 [60].
Test Type | Test Name | Biomarkers Targeted in SARS-CoV-2 | Sample Types | Time Taken for Result | Maker | References | |
---|---|---|---|---|---|---|---|
RT-PCR | Accula SARS-CoV-2 Test | N | Throat swab, Nasal swab | ~30 min | Mesa Biotech | [121] | |
RT-PCR | Xpert Xpress SARS-CoV-2 test for use on GeneXpert Xpress System | N2, E | NP swab, Nasal swab, mid-turbinate swabs, OP swab | ~40 min | Cepheid | [122] | |
Isothermal DNA amplification | Cue COVID-19 Test | N | Nasal swabs | ~20 min | Cue Health | [123] | |
Isothermal DNA amplification | ID NOW COVID-19 test | RdRp | NP swab, OP swab | < 13 min | Abbott | [124] | |
Molecular Diagnostics Tools for COVID-19 with FDA-EUA Approval. | |||||||
RT-PCR | 2019-nCoVReal-Time RT-PCR Diagnostic Panel | N1, N2, RP | NP swab, OP swab, Sputum, Tracheal aspirates, BAL, Nasal aspirate | ~80 mins | CDC | [125] | |
RT-PCR | QIAstat-Dx\ Respiratory SARS-CoV-2 Panel | ORF1b, E | NP swab | ~1 h | QIAGEN | [126] | |
RT-PCR | PerkinElmer® New Coronavirus Nucleic Acid Detection Kit | ORF1ab and N | NP swab, OP swab | ~2 h | PerkinElmer | [127] | |
RT-PCR | TaqPath COVID-19 Combo Kit | ORF1b, S, N | NP swab, BAL, Nasal swabs, OP swabs, Nasal aspirate, Mid-turbinate swabs | ~3 h | Thermo Fisher Scientific | [128] | |
RT-PCR | cobas SARS-CoV-2 | ORF1a/b, E | NP swab, OP swab | ~3 h | Roche | [129] | |
RT-PCR | Alinity m SARS-CoV-2 Assay | RdRp, N | NP swabs, OP swabs, BAL | ~2 h | Abbott Molecular | [130] | |
RT-PCR | BD SARS-CoV-2 Reagents for BD MAX System | N1, N2, | NP swab, OP swab | ~3 h | Becton, Dickinson & Company | [131] | |
RT-PCR | SARS-CoV-2 Assay | Not mentioned | NP swabs, OP swabs | NA | Gnomegen | [132] | |
RT-PCR | A rapid, automated in vitro real-time RT-PCR test | Nsp2 gene and N gene |
NP swabs, OP swabs | ~80 mins | NeuMoDx Molecular™ | [133] | |
RT-PCR | Only detects the COVID-19 | Not mentioned | NP swabs, OP swabs | NA | Primer design Ltd. COVID-19 genesis Assay | [134] |
Another possible approach for the inactivation of SARS-CoV-2 is photocatalytic inactivation. Titanium dioxide (TiO2), considered inert with low toxicity and nonsusceptibility to photo corrosion, exemplifies photocatalytic properties when illuminated under UV light [93]. Han et al. reported and tested the efficacy of Photocatalytic Titanium Apatite Filter (PTAF) over the inactivation effect on SARS-CoV. It was able to decompose the virus significantly with 6 h interaction under non-UV irradiation as well as UV irradiation. These photocatalysts when coupled with UV light can damage the spike proteins and decrease the infectious capability of the virus and thus can be helpful against SARS-CoV-2 [94].
4.2.4. Nanodelivery of Engineered Biomimetics: Artificial Viruses in the Making
Biomimetic solutions, generated from acumens from the natural world, may provide creative natural solutions [95]. Biomimetic approaches are now rapidly progressing towards the development of perfect carriers. With this approach, improved functional properties can be conferred by exploiting biological molecules, organisms and cells, or taking inspiration from them [13, 95]. Therefore, the governing principles of biomimicry and bioinspiration are implemented to design and engineer drug-delivery technologies for better functionality. The key success of the drug delivery strategy is highly dependent on the surface recognition and nanoscale interactions between the materials and biological building block's membrane proteins to convey the targeting [96]. Global attention has been given to the virus-based mode of operation by designing and creating virus-like nanoparticles which can circulate in the bloodstream by overpowering the endothelial barrier and delivering the therapeutic cargo with greater efficacy.
4.2.5. Development of Nanodecoys
Artificially developed decoys keep viruses at bay from human cells and can prove as another innovative way for managing viral infections. When a viral infection onset, the virus efficiently latches itself onto receptors of host cells and then replicates inside these cells. The “nanodecoys” are the same size as host cells possessing the same receptors which allow the viruses to “dock”. However, once the viruses are locked inside these nanodecoys their replication is prevented and thus their infectivity is curtailed. In this context, Rao et al. have developed a biomimetic nanodecoy using gelatine nanoparticle core camouflaged by mosquito host cell membrane to trap, divert and inhibit Zika virus infection in the mouse model [97]. As a therapeutic intervention for COVID-19, the same group developed a nanodecoy by fusing cell membrane nanovesicles obtained from human monocytes and genetically engineered cells expressing ACE2 receptors. These nanodecoys efficiently adsorbed SAR-CoV-2 viruses and inflammatory cytokines such as IL-6 and GM-CSF thus controlling the aggressiveness of viral infection and its related immunological issues [98].
4.3. As Control and Prevention Methods
4.3.1. Development of Vaccine and Nanomaterial-Based Vaccine Immunomodulation
Vaccination has been an effective medical intervention for the stimulation of immune response against infectious diseases [99]. Engineered nanoparticles have proven to have immunostimulatory effects, hence considerable attention can be given to the development of nanobased vaccines against different types of coronaviruses [100]. The creativity of nanoplatforms offers a great opportunity to incorporate antigen-presenting systems. Viruses with time undergo random mutation, which alters the antigen shape. In this regard nanotechnology offers the scope for functionalization with a wide number of signature molecules that can target the virus with altered shape to enhance the efficiency of the vaccine and prevent viral infection. Over the past decades, in the field of vaccine development, mRNA has been considered a potentially promising therapeutic tool [101]. In this context, therapeutic mRNAs-based vaccination has gained momentum in the nanotechnology-enabled strategies for cargo delivery [102]. Lipid encapsulated mRNA (mRNA-1273) nanovaccine, which encodes full-length, prefusion stabilized spike (S) protein of SARS-CoV-2 have enrolled in phase I clinical trial [103]. The subjects (> 18 years of age and non-pregnant female who are in good health) will receive an intramuscular (IM) injection (0.5 millilitres) of mRNA-1273 on 1st day and 29th day in the deltoid muscle and second vaccination after 12 months (day 394) with designed designated follow up for weeks. Similarly, anti-viral RNA vaccine in lipid polymer for active immunization against COVID-19 to be administered as intramuscular injection are being developed by companies like CureVac and BioNTech (in partnership with Pfizer) and are already in phase I/II clinical trials [103]. Further, one more adjuvanted inactivated vaccine based on aluminium salt (alum, also a nanomaterial) developed by Sinovac Biotech Co., Ltd, has illustrated partial or complete protection in rhesus macaques and is presently under Phase I/II clinical trials [68, 104-107]. The COVID-19 pandemic offers a scope and potential opportunity for nanotechnology-based vaccine adjuvant development. The idea of “nanoimmunity by design” mostly depends on the coherent design of nanomaterials with different physicochemical characteristics along with its scope for specific functionalization and fine-tuning its potential effect on the immune system [108]. Consortia and several groups are studying, characterizing, and screening nanomaterials according to their immunomodulatory properties and their cytotoxic effect for the development of adjuvants for clinical use. Moreover, the development of adjuvant is a lengthy process and generally takes several years [109]. Pharmaceutical giant GlaxoSmithKline (GSK) along with several partners is currently engaged in embedding their adjuvant systems with SARS-CoV-2-protein-based vaccines. Recombinant full-length SARS-CoV-2 glycoprotein nanoparticle vaccine adjuvanted with the saponin-based Matrix-M has been developed by Novavax and is currently under Phase I clinical testing [110]. Various statuses of different nanomaterial-based vaccine adjuvants have been reviewed [111, 112]. Numerous nanomaterials have demonstrated immunomodulatory effects and innate immune signaling [112]. Nanomaterial graphene oxide (GO) can provoke an inflammasome sensor (NLRP3)-dependent expression of IL-1β in macrophages [113]. Notably, alum, the most commonly utilized adjuvant in human vaccine induces cytokine production in macrophages through NLRP (Nod-like receptors-, LRR- and pyrin domain-containing protein) induced mechanism, suggesting that alum and GO can be useful for biomedical application [114]. Well, it is a known fact that vaccine development takes a long period to get approved and general application, in this context there is an urgent need for upgrading the prophylactic approach.
4.3.2. Nanotechnology as the New Foundation Towards Sustainable Health
The stability and behaviour of SARS-CoV-2 are different in temperature, pH, and UV light. However, it is found to be very stable at 25 °C and 4 °C. Apart from that, human coronaviruses can be deactivated by traditional sanitizers such as ethanol, bleach, povidone-iodine, chloroxylenol, chlorhexidine, and benzalkonium chloride [115]. SARS-CoV-2 persists till 72 h after inoculation on stainless steel and plastics and is deactivated on copper in less than 4h and on cardboard by 8h [116]. Also, the stability of the virus is dependent on the composition of the infected material as it can be inactivated in less than 3 h in printed and tissue paper, less than 2 days in treated wood and cloths, less than 4 days on banknotes and glass, less than 7 days on plastic and stainless-steel [117]. The virus can be active for more than 7 days on the outer layer of the surgical mask [118]. Information gathered in this regard, can be taken into consideration for the nanotechnology-based solution as an alternative method for classical chemical (hydrogen peroxide stream or metal-ion-coated surfaces), biological (probiotics or biosurfactants), and irradiation (UV) disinfection protocols used in healthcare settings. With the aid of nanotechnology, the development of pathways towards self-disinfecting surfaces that would evade infection of the healthcare and housekeeping staff is in process. The virus inactivation could be programmed with metal-based nanoformulations having intrinsic antipathogenic properties like GO, or having the ability to inactivate fungi and yeasts, bacteria, and viruses either photothermally or via photocatalysis induced ROS generation. As SARS-CoV-2 viral envelope proteins are damaged on Cu surfaces due to the generation of ROS, hence using copper and copper coating alloys base nanostructured surfaces rather than stainless steel (doorknobs, bed rails, etc.) would provide the antimicrobial effect. Copper oxide (CuO) impregnate mask was highly effective under simulated breathing conditions for (H1N1 and H9N2) viruses. The performance of its activity towards SARS-CoV-2 should also be investigated [119, 120].
CONCLUSION
The present pandemic situation involves the healthcare sector and requires a lot of introspection to comprehend the pathogenesis of SARS-CoV-2. With no absolute antiviral therapy, researchers are now focussing on alternative options. It is now evident that nanotechnology provides enough opportunities for diagnosis and therapy to tackle this critical situation. PPE, viral disinfectants, masks designed using nanotechnological know-how have an upper hand over their conventional counterpart. Highly specific and sensitive nanobased sensors have boosted the diagnosis scenario of the SARS-CoV-2 virus. Similarly, as therapeutics efficient antiviral delivery strategies should be designed in such a manner that they should be able to accomplish, sustained release, prolong circulation with less frequent administration, avoidance of opsonization, reduced therapy cost, minimal side effects, etc. In this scenario, all possible efforts to combat viruses based on currently available research outcomes can be applied towards the development of biodegradable, biocompatible, and non-toxic nanotechnology-based formulations harbouring these antivirals. This would bring an innovative perspective to convert conventional medicine for the inhibition of virus internalization or treatment. Besides, the development of a nanobased vaccination to aid the immune system has also garnered much attention. These nanotechnological weapons ameliorated with the knowledge of artificial intelligence will take the forefront for providing sustainable treatment on a global scale. However, more studies are necessary to understand the interface between nanoparticles and SARS-CoV-2, so that a rational design of targeted therapeutics can be achieved. All the products like PPE or masks must be evaluated for their side effects, such as skin irritation or allergy in humans. Besides, it has also come to the notice that nanoparticles can be released from these products as wastes to the environment during washing. Therefore, adequate measures must be taken to appropriately recycle them to avoid negative environmental impacts. If all these measures are considered properly, the day is not far when nanotechnology will be the first choice to devise management strategies for such pandemics.
CONSENT FOR PUBLICATION
Not applicable.
FUNDING
This study was supported by the Department of Science and Technology, Government of India, with grant [SR/WOS-A/LS-448/2017(G)] in the form of a women scientist fellowship (WOS-A).
CONFLICT OF INTEREST
The authors confirm that this article’s content has no conflicts of interest.
ACKNOWLEDGEMENTS
FD gratefully acknowledges the Department of Science and Technology, Government of India, for the financial support. RM thanks Sathyabama Institute of Science and Technology, Chennai for the support. SA is grateful to KIIT, Bhubaneswar for support.